- 1Division of Genetics and Development, Krembil Brain Institute, University Health Network, Toronto, ON, Canada
- 2Institute of Medical Science, University of Toronto, Toronto, ON, Canada
- 3Division of Neurosurgery, Krembil Neuroscience Centre, Toronto Western Hospital, University Health Network, Toronto, ON, Canada
- 4Division of Neurosurgery and Spine Program, Department of Surgery, University of Toronto, Toronto, ON, Canada
Abstract
Traumatic spinal cord injury (SCI) is a devastating and complex condition to treat with no curative options. In the past few decades, rapid advancements in our understanding of SCI pathophysiology as well as the mergence of new treatments has created more optimism. Focusing on clinical translation, this paper provides a comprehensive overview of SCI through its epidemiology, pathophysiology, currently employed management strategies, and emerging therapeutic approaches. Additionally, it emphasizes the importance of addressing the heavy quality of life (QoL) challenges faced by SCI patients and their desires, providing a basis to tailor patient-centric forms of care. Furthermore, this paper discusses the frequently encountered barriers in translation from preclinical models to clinical settings. It also seeks to summarize significant completed and ongoing SCI clinical trials focused on neuroprotective and neuroregenerative strategies. While developing a cohesive regenerative treatment strategy remains challenging, even modest improvements in sensory and motor function can offer meaningful benefits and motivation for patients coping with this highly debilitating condition.
Impact statement
Despite advancements in medical, surgical, and rehabilitation management for traumatic spinal cord injury (SCI), there remains a critical need for neuroprotective and neuromodulatory treatment strategies. By providing an overview of the current state of SCI understanding and management strategies, this paper aims to bridge the gap between current therapeutic limitations and emergent treatments. It also examines the challenges in treating and studying SCI due to the complexities in the heterogeneity of the disease. Emphasizing the integration of patient feedback and emergent therapies, this paper advocates for the development of tailored approaches that are crucial for advancing SCI care and inclusivity. Ultimately, the goal is to provide insights and guidance that will enhance recovery and quality of life outcomes for SCI patients, benefiting researchers, healthcare professionals, policymakers, and caregivers alike.
Introduction
Traumatic spinal cord injury (SCI) remains a debilitating condition, but over the past century rapid growth has been made to uncover its pathophysiology and translate preclinical research to patient care. This paper provides an overview of SCI pathophysiology, epidemiology, currently employed management strategies, and emerging therapeutic approaches. It also highlights the quality of life (QoL) challenges faced by patients as well as their desires, providing a basis for caretakers to tailor more patient-centric forms of care. This review underscores the heterogeneous nature of SCI both in disease presentation and individual patient needs, having profound effects on treatment effectiveness. By delving into the wide range of strategies to manage SCI, both established in the clinic and emerging approaches, this paper examines their therapeutic potential and limitations. Furthermore, this paper discusses the frequently encountered barriers in translation from preclinical models to clinical settings. Although the need remains urgent for novel and effective SCI treatments, there is great hope with the continued progress in the field aimed at enhancing QoL and functional outcomes for patients.
Epidemiology
Of those that survive the initial injury, most will have persisting neurological deficits [1]. Direct costs incurred by SCI due to permanent disability are large, estimated to be between 1.1 and 4.6 million USD per patient in the United States [2]. The World Health Organization estimates that 250,000 to 500,000 people suffer a new SCI each year [3] but direct comparisons are shrouded by a lack of an international standard for SCI reporting. Despite challenges surrounding SCI reporting, commonalities can still be drawn from regionally reported data. Within developed countries, SCIs are primarily caused by motor vehicle accidents (MVAs), yet there is a shift towards an increase in fall-related injuries [1, 4, 5]. For example, in the United States, 38.1% of injuries were caused by MVAs from 2010 to 2014, with falls as the close second cause at 31.0% [6].
In regard to sex, males make up the highest distribution of SCI at 79.8% as opposed to female SCI cases at 20.2% [6]. Within the elderly population, this disparity between sex decreases as the age at which SCI occurs in females tends to be later [7, 8]. Preclinical models for SCI assessing the role of gonadal hormones do not have an established consensus [7]. Clinically, the higher incidence of male SCI as well as disparities in the cause and types of injuries make sex-based comparisons difficult.
With an aging global population, the average age of injury is increasing from patients in their late 20s to those in their early 40s [2]. This increase in age remains true for most causes of SCI, with the exception of violence, as it predominantly occurs in younger individuals (16–30 years of age) [6]. In comparison to younger patients, individuals over 50 have greater rates of cervical injury leading to paraplegia than their younger counterparts [9].
There are also variations in injury trends between countries, predominantly related to economic status. Developing countries primarily report falls as the leading cause of SCI, while MVAs dominate SCI cases in wealthier nations [5, 10–12]. However, there are exceptions to this trend. Prevention efforts have reduced MVAs, work-related SCIs, and driving-related injuries in high-income countries. Unfortunately, MVAs and work-related SCIs remain significant issues in low and middle-income countries. Advances in acute surgical, medical, and rehabilitation care have disproportionately benefited high-income countries [5, 10–12].
Despite its stronger economic position in the world, falls are beginning to dominate the SCI landscape in Japan due to the large elderly population [13]. Violence also contributes to a greater proportion of SCI cases in developing regions [14]. Even within a nation, variations in urbanization, economic status, and occupation have different outcomes [15].
Mortality and quality of life in patients with SCI have improved but remain lower than in healthy, age-matched controls in the global population [16]. In the first-year post-injury, the mortality rate is close to 3.8%, followed by 1.2% the next year and an increased rate of 1.2% per annum over the next 10 years [16]. The most significant indicators of mortality in the time surrounding the injury are the severity of the SCI as well as the level of the SCI and the age of the patient [16–18]. Major risks that consistently place patients at higher long-term mortality rates are a loss of autonomy as well as reduced social engagement and support [19, 20].
Pathophysiology
SCI is a heterogeneous and multifaceted condition that threatens the physical, social, and vocational well-being of patients. It is one of the leading causes of paralysis worldwide [1, 21]. SCI begins with an external mechanical trauma that causes contusion and compression of the spinal cord (Figure 1). This leads to the generation of toxic debris and disruption of vasculature, which initiates the secondary injury cascade [22]. In the acute phase (<48 h post-injury), inflammation is initiated accompanying the activation of microglia into a proinflammatory phenotype, which leads to glutamate excitotoxicity and nitric oxide production [1, 23, 24]. Furthermore, blood-spinal cord barrier (BSCB) disruption, hemorrhage, ischemia, as well as demyelination contribute to greater neuronal and glial cell death [25, 26]. The subsequent subacute phase (2–14 days post-injury) sees sustained inflammation and ischemia as well as the recruitment of resident astrocytes into reactive astrocytes [27, 28]. These astrocytes have impaired glutamate reuptake contributing to excitotoxicity, disrupted BSCB contact and maintenance, as well as formed chondroitin sulfate proteoglycan (CSPGs) deposits that disrupt regeneration [25]. Ependymal cells undergo significant alterations after SCI. This involves activating specific signaling pathways in the spinal cord that promote self-renewal, proliferation, and differentiation. An orchestrated regulation of receptor and ion channel expression fine-tunes and coordinates the activation of ependymal cells after SCI or cell transplantation [29]. While ependymal cells have been proposed as adult neural stem cells, controversy remains as to whether they provide a significant portion of scar-forming astrocytes to protect tissue and function after SCI [30]. Infiltrating microglia is also a key cellular component in orchestrating the glial scar that develops after SCI to protect neural tissue [31].
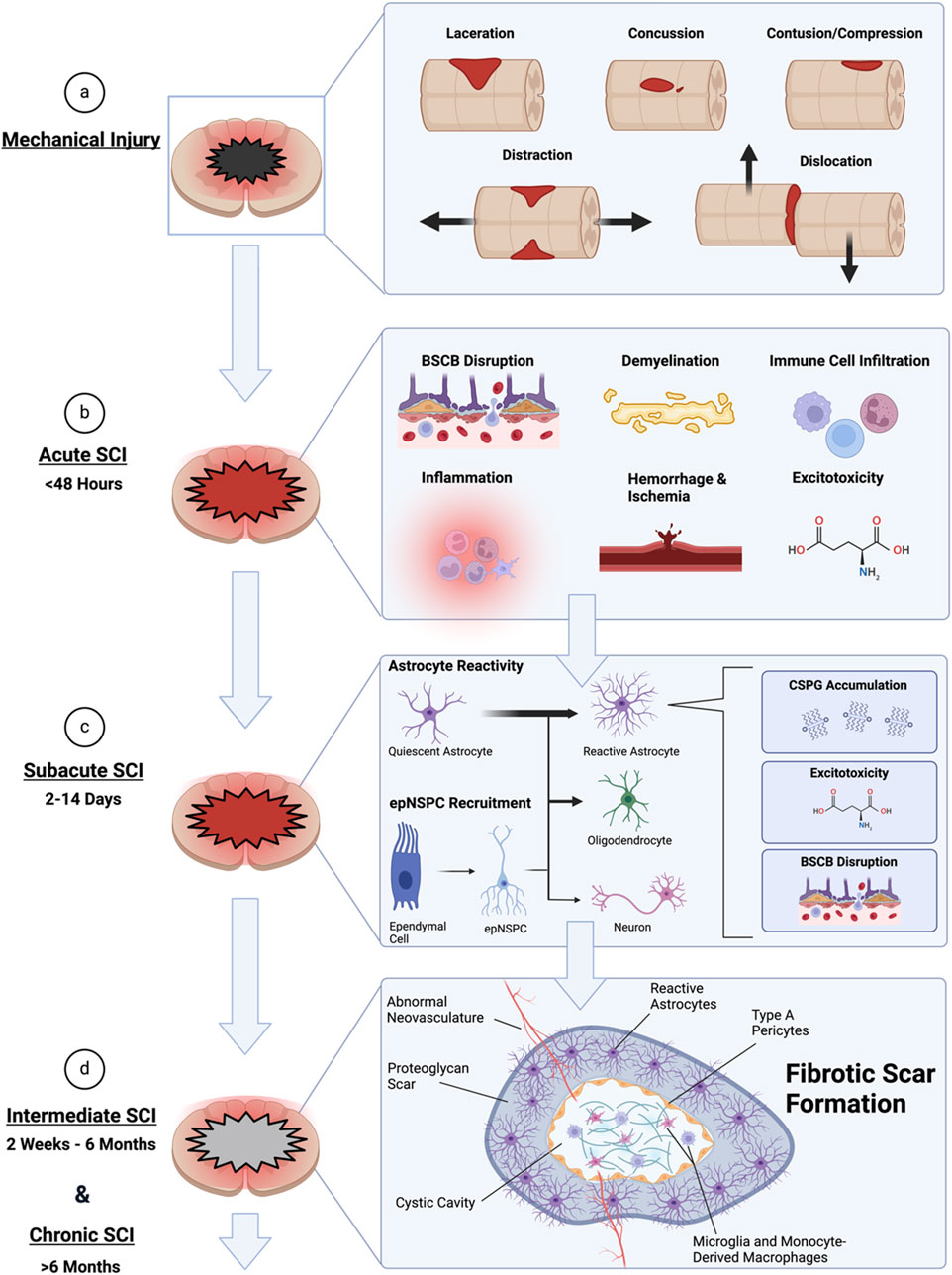
Figure 1. Timeline of the pathophysiological developments of spinal cord injury (SCI). (A) The initial mechanical forces that contribute to lesion formation and necrosis, initiating SCI. (B) The acute phase of injury occurs following the initial injury. It is characterized by inflammation, ischemia, blood-spinal cord barrier (BSCB) disruption, immune cell infiltration and recruitment, demyelination, as well as excitotoxicity. This leads to further damage of the parenchyma beyond the initial lesion. (C) The subacute phase sees the recruitment of astrocytes from their quiescent state to reactive. Astrocytes are also derived from resident ependymal cells through neural stem/progenitor cell (epNSPC) differentiation. Predominantly epNSPCs differentiate into astrocytes, with few becoming oligodendrocytes and even less becoming neurons. The reactive astrocytes then contribute to further disruption of the BSCB, reduced glutamate uptake involved in excitotoxicity, and chondroitin sulfate proteoglycan (CSPG) deposition. Inflammation and ischemia also persist in this phase. (D) During intermediate and chronic phases of SCI, the reactive astrocyte border and fibrotic scar is formed and consolidated. The fibrotic scar contains type A pericytes, abnormal vasculature growth, and CSPG deposits. The scarring and cystic formation inhibits recovery. Created with Biorender.com.
Resident ependymal cells are recruited during this period and form neural stem/progenitor cells (epNSPCs) that predominantly differentiate into astrocytes [32, 33], contributing to the upregulated population of reactive astrocytes. In the intermediate (2 weeks-6 months post-injury) and chronic phases of SCI (>6 months post-injury), a fibrotic scar core consisting of type A pericytes, abnormal neovasculature, and CSPGs is formed within a reactive astrocyte encasing border [34]. Scarring, cystic cavity formation, as well as limited remyelination and axon regrowth act in concert to greatly stunt recovery. This causes devastating and often permanent neurological deficits with complex barriers to treatment.
Targets for potential SCI treatment
BSCB disruption
The BSCB is a special structure within the spinal cord parenchyma that mediates the exchange of compounds between the blood and the parenchyma while maintaining a regulated chemical balance and homeostasis crucial for neural function [35–37]. Preserving the integrity of the BSCB may enhance spinal cord repair and functional improvement, therefore, the BSCB plays a role in the pathophysiology of SCI progression [34–36].
Morphological and functional changes in the BSCB after SCI include vascular changes, increased permeability of the BSCB, edema, and cavity formation [38]. The initial mechanical damage, combined with compression, laceration, and distraction, contributes to disruption of the neurovascular system [35]. The adverse environment then rapidly results in neuropil damage, swollen neurovascular unit cells, and membrane structure disruption [32, 36, 37]. The morphological alterations accompanying BSCB disruption are instrumental in the progression of SCI because the disrupted BSCB allows the immune cells to enter the injured sites [35, 39, 40].
Lymphocytic infiltration mediates inflammation, reactive astrogliosis, scar formation, and neutrophils leading to demyelinating as well as neuroinflammatory events [39, 40]. The BSCB alterations following SCI lead to altered permeability, which commences several minutes after injury, persists for up to 4 weeks, and may extend over a longer duration, often accompanied by cavity formation [41, 42]. In addition, following SCI, edema begins within several minutes, intensifies rapidly, and persists for up to 15 days, affecting both the lesion site and adjacent segments [38, 39]. Progressive cavity formation causes deficits in neurological function and neuropathic pain [43–45].
Inflammation
Although inflammation serves as a vital defense mechanism in removing pathogens, clearing debris, and facilitating wound healing in the context of SCI, it also accentuates detrimental effects [45, 46] Following SCI, the inflammatory response leads to the production of toxic molecules which, instead of aiding in healing, cause further damage on otherwise intact tissues. While inflammation is required for repair, the response that follows SCI is often exaggerated and leads to further damage and cell loss [47]. Further adding to the complexity of SCI, the infiltration of immune cells non-resident to the CNS (central nervous system) also plays a critical role in inflammation and signaling molecules, affecting the progression of the disease. These infiltrating immune cells are guided by cytokines produced from astrocytes, microglia, peripherally derived macrophages, and endothelial cells [48, 49].
Following SCI, Microglia change their cellular morphology and protein expression profiles [49–51]. Under normal physiological conditions, microglia have long, thin processes that extend from the central cell body to sample the extracellular environment [50, 51]. After SCI, microglia retract their processes and assume an amoeboid shape, primed for phagocytosis and debris clearance [49–51]. In the first hours after injury, microglia, astrocytes, and neurons synthesize pro-inflammatory cytokines [49, 52]. Chemokines drive the increased expression of selectins and cell adhesion proteins on endothelial cells, facilitating integrin-mediated adhesion of circulating immune cells and the subsequent leakage of monocytes and neutrophils into the spinal cord [53, 54]. As the injury response progresses, microglia proliferate extensively during the first 2 weeks, accumulating around the lesion site. These activated microglia position themselves at the interface between infiltrating leukocytes and astrocytes, orchestrating glial scar formation by releasing factors such as IGF-1 [31].
Infiltrating macrophages provide proteolytic enzymes, reactive oxygen species, and inflammatory cytokines to the injury microenvironment but also perform the necessary functions of debris clearance, cellular remodeling, and producing pro-regenerative factors [55, 56]. Preclinical studies have shown that while macrophages increase axon regeneration and neuronal function, they can also worsen tissue destruction. The dual beneficial and reparative functions of macrophages make understanding their role in the injury response difficult [55, 56].
Ischemia and hemorrhage
Mechanical damage from SCI leads to the disruption of capillaries and the BSCB, which creates a harsh microenvironment for spinal cord parenchyma [57]. A direct rupture of the local capillaries induces bleeding into the parenchyma of the spinal cord, which could cause increased release of cytokines and chemokines from macrophages, microglia, and astrocytes into the extracellular space [57]. The presence of red blood cells in the parenchyma is likely to induce free radicals and consequently lead to edema [58, 59]. On the contrary, neural tissue edema can also increase interstitial pressure, which presses the neighboring vessels and causes ischemia [60]. The lack of adenosine triphosphate caused by ischemia and ion channel defects results in an ion imbalance [60, 61].
Demyelination and re-myelination
Oligodendrocytes oversee the generation and maintenance of myelin segments, which is crucial to maintaining the integrity of axons and eases axon signal conduction [62, 63]. After SCI, mechanical damage and the imbalance of local microenvironment factors leads to demyelination [64, 65]. The apoptosis of oligodendrocytes is potentially the leading cause of axonal demyelination [64, 65]. The level of oligodendrocyte apoptosis at the epicenter of the lesion peaks within a week of the injury; however, uninjured axons around the lesion remain myelinated [64, 65]. The presence of myelin debris inhibits remyelination, thus the extent and quality of remyelination are limited [66].
Mechanical injury, ischemia, inflammatory cytokines, oxidative stress, excitotoxicity, and autophagy can cause the death of oligodendrocytes because of demyelination and remyelination imbalance [67–69]. Molecules involved in demyelination are potential inhibitors of axon regeneration, thus the process of demyelination inhibits the regeneration of axons [67–69].
Following a SCI, remyelination mainly involves replacing oligodendrocytes, with the primary source of these new cells being progenitor oligodendrocytes and endogenous neural stem cells [67]. Endogenous neural stem cells remain inactive in normal conditions and become activated upon spinal cord damage; these cells mainly differentiate into astrocytes and to a lesser degree into oligodendrocytes [67, 70]. This suppression of differentiation into oligodendrocytes is mainly due to the lack of growth factors that switch the balance toward differentiation into oligodendrocytes [67, 70].
Hyperexcitation (switch from KCC2 to NKCC1)
NKCC1 and KCC2 are members of the SLC12 cation-chloride co-transporter (CCC) family, which participate in physiological and pathophysiological processes by regulating intracellular and extracellular chloride concentrations, and in turn the GABAergic system [71, 72]. NKCC1 transports Cl− into cells while KCC2 transports Cl− out of cells, thereby regulating chloride balance and neuronal excitability. An imbalance of NKCC1 and KCC2 after SCI will disrupt CI− homeostasis, resulting in the transformation of GABA neurons from an inhibitory to an excitatory state, which leads to abnormal conditions such as spasticity and neuropathic pain [73–75].
After SCI, the segment below the injury site presents a state similar to upregulation of NKCC1 seen in the early stages of development [75]; therefore, the expression of KCC2 was reported to be downregulated at the injury site, followed by a transient upregulation of NKCC1 expression levels, and this altered expression trend was consistent with the post-neuropathic pain occurrence [76].
Inflammation or injury can inhibit the expression and function of KCC2 in the dorsal horn and advance the development of neuropathic pain [77, 78]. GABAA receptors (GABAARs) are involved in the regulation of tonic inhibition in the dorsal horn, sustaining the relative balance of inhibition and excitation in the central nervous system [79]. After SCI, the function of GABAARs changes and their activation can cause a depolarizing shift as well as the disclosure of nociceptive sensitization [80]. Therefore, improving the abnormal Cl− concentration gradient in the dorsal horn through targeting KCC2 and NKCC1 represents a promising therapeutic direction for restoring the inhibitory function of the GABAergic system and relieving or improving neuropathic pain [81–83]. Moreover, disruption of Cl− homeostasis after SCI, especially the downregulation of KCC2 in motor neurons, depolarizes the Cl− equilibrium potential and decreases the strength of postsynaptic inhibition [74].
Numerous studies have confirmed the therapeutic effect of NKCC1 and KCC2 in neuropathic pain, spasticity, and motor functional recovery post SCI, and these co-transporters are expected to become key targets in future SCI treatments [74, 84]. However, KCC2 and NKCC1 are distributed throughout the nervous system and methods to achieve localization, orientation, and quantitative regulation of their levels may be the main obstacle to their clinical application in the treatment of SCI.
Patient-centric approaches
The concept of patient-centered care (PCC) is crucial for bridging the gap between patients’ desires and what healthcare professionals consider beneficial for patients. Gerteis et al. identified that patients defined PCC as having the following dimensions: 1) respect for patient’s values, preferences and expressed needs; 2) coordination of care and integration of services within an institutional setting; 3) communication between the patient and providers; 4) dissemination of accurate, timely and appropriate information; 5) education about the long-term implications of disease and illness; 6) physical care, comfort and the alleviation of pain; 7) emotional support and alleviation of fears and anxiety; 8) involvement of family and friends; and 9) transition and continuity from one locus of care to another [85]. This section will delve into specific dimensions of PCC relevant to patients with SCI, with a focus on translational perspectives.
Respect for patients’ desires
Healthcare professionals generally strive to provide the best treatment for patients with SCI in accordance with clinical practice guidelines [86]. However, therapeutic strategies for SCI do not always align with patient satisfaction. According to a qualitative study on decision-making regarding bladder drainage methods after SCI, conducted by Engkasan et al., some patients felt that they were forced to accept their doctor’s decision [87]. Additionally, Scheel-Sailer et al. reported in their qualitative interview-based study that patients with SCI often experience difficulties making decisions during the initial rehabilitation phase due to physical, psychological, and environmental factors [88]. Thus, it appears that patients’ opportunities for decision-making in therapeutic strategies for SCI might be limited in certain contexts.
Importantly, patients’ treatment preferences might differ from the actual treatments, potentially undermining respect for their desires. Bowers et al. conducted a survey to clarify SCI patients’ preferences regarding methylprednisolone sodium succinate (MPSS) treatment for acute SCI and found that most SCI patients considered MPSS treatment important, even if it offered only minor neurological benefits and carried a risk of complications [89]. However, the 24-hour administration of high-dose MPSS to adult patients within 8 h of SCI is still controversial, with only a weak recommendation in the 2017 AO Spine Clinical Practice Guidelines [86]; therefore, not all patients wishing to receive this treatment may be able to, depending on the physicians’ decision.
Looking forward, as novel therapeutic strategies for SCI emerge, they will initially lack robust evidence to guide evidence-based decision-making. In such situations, it will be important for physicians to respect patient’s desires and provide them with opportunities to make decisions about their treatments.
Accessibility of information
Patients with SCI have a keen interest in health-related information; hence, the accessibility of such information is crucial for them [90]. According to the interest assessment survey performed by Edwards et al. in the early 2000s, 64% of Canadian chronic SCI patients reported using the Internet to obtain research information [91]. In a more recent 2020 study by Farrehi et al., 89% of participants with SCI in the United States reported sourcing information about experimental therapies online [92]. This trend suggests that access to medical information, including emerging therapies, will continue to grow in the future. However, SCI patients tend to deem information from SCI specialists as more reliable [92]. Therefore, it is equally important to enhance accessibility to SCI specialists, and there are opportunities to leverage emerging areas, such as Telemedicine, to improve access for patients in rural areas [93].
Moreover, improving accessibility for research information also benefits researchers by aiding in the recruitment of participants for clinical trials, as individuals with SCI are willing to participate in translational research [94].
Enhancing quality of life for patients
Among the various neurological symptoms experienced after SCI, pain is the most prevalent issue of SCI as highlighted by patient feedback, followed by bowel and bladder dysfunction, spasticity, and sexual dysfunction [95, 96]. It significantly affects patients’ quality of life by interfering with sleep and daily activities [95]. A survey by Jensen et al. found that pain was both the most common (experienced by 84% of individuals) and the most severe symptom among participants [96]. According to a meta-analysis regarding the prevalence of neuropathic pain following SCI, the pooled point prevalence rate was 53% [97]. However, a postal survey by Finnerup et al. indicated that only a small number of patients received treatment with antidepressants or anticonvulsants, which are considered to be most effective for neuropathic pain [98]. This suggests that there is significant potential for enhancing the quality of life in SCI patients.
In preclinical studies, behavioral assessment tests for sensory function are less commonly utilized than those for motor function. A systematic review regarding animal models of SCI showed that sensory tests, such as the von Frey filament test, were used in only 16.3% of studies, compared to 89.2% for locomotor tests [99]. This discrepancy might be due to most researchers focusing primarily on motor function. However, considering the clinical application and the impact on patients’ quality of life, there may be merit in the inclusion of sensory assessment as well as locomotor assessment.
Currently employed strategies in the management of SCI
Early surgical decompression
Surgical decompression of the spinal cord within the first 24 h after injury limits tissue damage by restoring compromised blood flow and reducing the extent of ischemia-related secondary injuries. A recent pooled analysis to evaluate the efficacy of early decompressive surgery for SCI demonstrated that the American Spinal Injury Association (ASIA) motor score in the early (within 24 h of SCI) surgery group was significantly higher than that in the late (after 24 h of SCI) surgery group (23.7 points vs. 19.7 points; p = 0.0006) at 1 year after injury [100]. According to the latest meta-analysis regarding timing of decompressive surgery for acute SCI, patients were 2 times more likely to recover by ≥2 grades on the ASIA Impairment Score at 6 months and 1 year after SCI (risk ratios: 2.76 [95% CI: 1.60–4.98] and 1.95 [95% CI: 1.26–3.18]) if they underwent decompressive surgery within 24 h after injury [101]. Based on this evidence, the recommendation for early surgical decompression (within 24 h after SCI) was upgraded in the recently published AO Spine-Praxis Clinical Practice Guidelines from “Quality of Evidence: Low; Strength of Recommendation: Weak” in 2017 [86] to “Quality of Evidence: Moderate; Strength of Recommendation: Strong” in 2024 [101]. Although the evidence has become stronger, early surgical decompression for acute SCI remains a significant challenge in low- and middle-income countries due to limited logistical and infrastructural resources [102].
As for ultra-early surgical interventions (within 4, 5, 8, and 12 h after SCI), it is difficult to draw firm conclusions on their efficacies compared to early surgical decompression, due to the inconsistency in results observed so far [101]. Just as the evidence for early surgical decompression has been established, the evidence for ultra-early surgical intervention is expected to be solidified as more clinical findings become available.
Recently, a phase III RCT, Duroplasty for Injured Cervical Spinal Cord with Uncontrolled Swelling (DISCUS) (NCT04936620), was initiated. This ongoing trial compares laminectomy with duroplasty to laminectomy alone for treating acute cervical SCI. It is expected to reveal the optimal surgical procedure for acute SCI in the near future.
Blood pressure augmentation
Hemodynamic management following acute SCI is crucial, as ischemia and hypoperfusion can exacerbate secondary injury. Pre-clinical research has indicated that maintaining arterial pressure can improve spinal cord blood flow and, consequently, electrophysiological function [103, 104]. Accordingly, the 2013 guidelines from the American Association of Neurosurgical Surgeons (AANS) and the Congress of Neurological Surgeons recommended maintaining a mean arterial pressure (MAP) of 85–90 mmHg for the first 7 days post-SCI [105]. However, considering the strict MAP target range of 5 mmHg and newer literatures since the 2013 AANS/Congress of Neurological Surgeons guidelines, the 2024 AO Spine Guideline now recommends that MAP should be maintained between 75 and 80 mmHg as a lower limit and not exceed 90–95 mmHg at the higher range during the first 3–7 days post-SCI [106]. A phase III, randomized, controlled trial (RCT), the Randomized Trial of Early Hemodynamic Management of Patients Following Acute Spinal Cord Injury (TEMPLE) (NCT02232165), was initiated in 2017. This ongoing trial aims to compare augmented blood pressure management (targeting MAP of 85–90 mmHg) with conventional management (65–70 mmHg), and may provide additional evidence on the benefit of blood pressure augmentation for acute SCI.
Additionally, spinal cord perfusion pressure (SCPP), which has recently emerged as a more relevant parameter to predict functional outcomes as compared to MAP, is recommended to be maintained above 50 mmHg [107]. It is anticipated that the ongoing Canadian-American Spinal Cord Perfusion Pressure and Biomarker Study (CASPER) (NCT03911492) will soon provide further evidence to support this approach.
Methylprednisolone sodium succinate (MPSS)
MPSS is a corticosteroid that inhibits lipid peroxidation of the neuronal membrane and prevents secondary damage of SCI [108]. The National Acute SCI Study (NASCIS) trials were representative trials of MPSS for SCI. In the NASCIS-2 trial, the primary analysis did not show significant motor recovery in the MPSS group; however, secondary analyses demonstrated that patients who had received high-dose MPSS within 8 h post-SCI improved motor scores compared to the control group at 6 months post-SCI (16.0 points vs. 11.2 points; p = 0.033) [109]. Additionally, the NASCIS-3 trial suggested that patients who received MPSS within 3 h post-SCI should be maintained on the 24-hour treatment regimen, whereas those who received 3–8 h after SCI should be maintained on the 48-hour therapy [110]. Although there are some controversies from a perspective of complications [111], the side effects of steroids are much less relevant in modern times with improved general medical care and the avoidance of steroids in medically compromised individuals. Currently, a 24-hour infusion of high-dose MPSS should be offered to adult patients with acute SCI (<8 h post-injury) as a treatment option [86].
Challenges in translation
Generally, the process from technology initiation to FDA approval in translational science takes a considerable amount of time. McNamee et al. reported that the median interval from technology initiation to establishment was 25 years, to the start of clinical trials was 29 years, and to the first FDA approval was 36 years among new molecular entities approved by FDA between 2010 and 2014 [112]. Broadly, clinical and translational research encompasses the following five phases: T0, basic research (pre-clinical research); T1, translating basic research to humans (phase I clinical trials); T2, translating findings to patients (phase II/III clinical trials); T3, translating research to general practice care (phase IV clinical trials); and T4, translating research to populations or communities [113]. This section will focus on animal models for phase T0, highlight key clinical trials for phase T1–2, and examples of advanced translation.
Animal models and clinical relevance
In translational research, multiple animal models should be used to verify the effectiveness of potential treatments and establish proof of concept. Utilizing a variety of models enhances the robustness and translatability of the research findings [114]. When considering differences among preclinical SCI models, it is important to note the animal species, injury mechanisms, and injured level.
Animal species
Rodent models are the predominant model in SCI research. Rats are most commonly used (72.4%) in SCI preclinical research, followed by mice at 16% [99]. Rodent models also have distinct phases of SCI pathophysiology that are clinically relevant and, given their rapid reproduction cycle and small size, can allow for greater sample sizes. This is especially useful in drug studies where multiple groups are needed to test a range of dosages for safety and efficacy. The preference for rat models is due to their long-term usage as a robust and reliable model for assessing even incremental improvements [115]. Immunodeficient rats that lack T-cell presence have allowed for cell transplantation therapy experiments without the risk of host-vs-graft disease. As an example, human pluripotent stem cell lines have been applied in these models with success [116]. The main caveat to this model, however, is that immunosuppression of SCI patients to allow for transplantation would be through immunosuppressant drugs rather than genetic alterations. This hinders translatability but also reduces variability from the many possible tailored immunosuppressant regimes. For more extensive genetically modified animal research, mouse models are utilized due to their widespread usage in knockout studies. Immunodeficient mice with engrafted human hematopoietic stem cells have shown promise as a translatable model for human immune responses after SCI [117]. However, they are less resilient to SCI induction with higher mortality rates and have species-specific timelines in SCI less reflective of human patient timelines. Where rats have T-cell infiltration peak at 3–7 days post-injury, closer to the human timeline of 7–9 days post injury [49], mice do not have significant T-cell infiltration until after 14 days post injury [49].
Despite the advantages of rodent models, there is a need for large animal and non-human primate models from a translational perspective [118, 119]. When comparing SCI in rodents and humans, functional recovery after injury in rodent models tends to be much faster compared to humans, which seems to be associated with various neural pathways. For example, the rubrospinal tract has been reported as an alternative pathway to improve motor function after corticospinal tract injury in rodent models, which is not observed in humans [120, 121]. Moreover, the size of the spinal cord and its surrounding environment, including the cerebrospinal fluid, differ considerably in rodents, potentially affecting the distribution of locally delivered therapies [122]. This holds especially true for the development of surgical interventions, which are limited when applied to the small stature of rodent models. By examining both rodent and large preclinical animal models, more robust findings can be obtained prior to clinical trials. To date, various large animal SCI models have been utilized, including those involving pigs [123], dogs [124], cats [125], and monkeys [126].
Injury mechanisms
Based on the mechanisms of injury, SCI models can be classified as contusion, transection, compression, and distraction/dislocation models. Contusion models are the most commonly used (43.4%), followed by transection (34.4%) and compression models (20.5%) [99].
Contusion models are created using weight-drop apparatuses or electromagnetic impactors, such as the New York University impactor [127] and the infinite horizon impactor [128]. Compression models are generated by compression using a modified aneurysmal clip [129], forceps [130], or balloon [131]. Both contusion and compression models effectively reflect the pathophysiology of SCI; in particular, clip contusion models not only cause compression but also contusion and hypoperfusion, closely mimicking clinical situations [118]. Transection models, which include complete and partial transection, are advantageous for investigating axonal regeneration following SCI. However, they do not fully represent the complex pathophysiology of SCI, as the spinal cord is seldom sharply transected in clinical settings [118].
Injured levels
Although approximately 60% of SCIs occur at the cervical level [132], only 12% of preclinical research studies have utilized cervical models, with the majority (over 80%) employing thoracic SCI models [99]. This discrepancy may stem from the challenge of postoperative care in cervical SCI models, including manual bladder expression and feeding as well as daily fluid administration, which is necessary to maintain low mortality rates [133]. While challenging to implement, it is essential to validate therapeutic effects in cervical models, as the pathophysiology of cervical SCI differs from that of thoracic SCI due to anatomical and physiological variations [134].
Even though the animal models can be sophisticated from a clinical relevance perspective, a large gap between preclinical studies and early-phase clinical trials remains due to issues of poor validity and reproducibility, often caused by improper study designs. The lack of alignment in design between basic research and clinical trials, including the dosage of drugs and timing of administration, makes it difficult to predict the effectiveness of novel therapies in human trials [135]. To maximize clinical translation, we should refine study designs as well as using clinically relevant animal models.
Regenerative strategies under research
Stem cells and associated growth factors
Endogenous stem cells
Cellular replacement strategies are necessary to restore disrupted neural signaling pathways following the extensive parenchyma loss after SCI. Oligodendrocyte progenitor cells (OPCs) are the majority of progenitor cells that proliferate and differentiate in response to SCI [136]. OPCs are unipotent and neural stem/progenitor cells (NSPCs) are fewer, underlying the spinal cord’s limited neurogenic potential [137]. Of the NSPCs, the largest population responding to SCI are ependymal derived neural/stem progenitor cells (epNSPCs) in the spinal canal. They display multipotent properties and are capable of self-renewal, responding to SCI in the acute phase through proliferation and migration to the site of injury. Although they have been shown in vitro to have the capability to differentiate into neurons, astrocytes, and oligodendrocytes, in vivo studies have displayed them to be particularly biased towards an astrocytic fate post-SCI, with a small potential for becoming oligodendrocytes and an even lower potential to form neurons [137, 138].
Strategies to bias epNSPCs towards neuronal cell fates are emerging. Bioartificial scaffoldings to bias NSPCs towards neuronal cells such as those developed by Zhang et al. [139] have been shown to facilitate neural stem cells to differentiate into neuron-like cells. As an alternative approach, biasing through changes to the microenvironment has also been displayed. Ohori et al. [140] injected fibroblast growth factor 2 and epidermal growth factor within the lesion site in a rat SCI model to promote immature markers of neuronal cells in NSPCs. As a caveat, they used NSPCs that were genetically manipulated by a retrovirus, pMXIG, to express Neurogenin2 (NGN2) and Mash1, transcription factors that bias towards neurons and oligodendrocytes.
Induced pluripotent stem cells
Induced pluripotent stem cells (IPSCs) can be biased as neural progenitor cells (NPCs) for transplantation and integration into the spared parenchyma to enhance local circuits and aid in motor recovery [141]. These cells can be made in an autologous fashion from fibroblasts, circumventing the need for immunosuppressants when using exogenous cells as well as the ethical concerns of their origins. Fibroblasts are exposed to the factors Oct4, Sox2, Klf2, and c-Myc in accordance with the work done by Yamanaka and Takahashi on mice in 2006 [142] and in human fibroblasts by Takahashi et al. in 2007 [143]. This comes at the cost of time, however, for the development and biasing of IPSCs, limiting their application to later stages of injury.
Generating IPSCs also comes with added risks, particularly related to tumorigenicity. When generating the IPSCs, residual undifferentiated cells can proliferate and form tumors [144], thus emphasizing the need for stringent quality control.
One of the factors used to generate IPSCs from fibroblasts, c-Myc, is protooncogenic and often overexpressed in a majority of human cancers, contributing to over 40% of tumor formations [145, 146]. Retroviral c-Myc introduction has also shown an increased tumorigenicity in mouse models [147]. Clinically this carries a large risk. Using alternative factors reduces the efficiency and speed of IPSC induction but makes the IPSCs clinically acceptable [148].
The first IPSCs generated by Yamanaka and Takahashi through retrovirus transduction resulted in random integration [142, 143] at start sequences with increased likelihoods of loss of function effects [149]. Alternative strategies have arisen with lowered tumorigenicity. Sendai and adeno-associated viruses, as well as plasmid integration, have been used to potentially lower teratogenicity but are highly inefficient [150–152]. Sustained delivery of synthetic mRNA that encodes for the reprogramming factors used by Yamanka’s group are more efficient and avoid the heritable tumorigenicity of cellular DNA modification [153, 154]. Direct reprogramming of adult somatic cells without viral vectors through the transient expression of Msi1, Ngn2, and MBD2 by Ahlfors et al. has shown high reprogramming efficiency, no tumorgenicity in murine models, and low-cost [155]. It remains hopeful for clinical application.
Overview of current significant clinical trials
In the currently employed therapies for SCI, there are not enough neuroprotective and neuro regenerative approaches. To address this issue, numerous clinical trials have been performed. In this section, we provide an overview of clinical trials examining neuroprotective (Table 1), regenerative (Table 2), and other strategies for SCI.
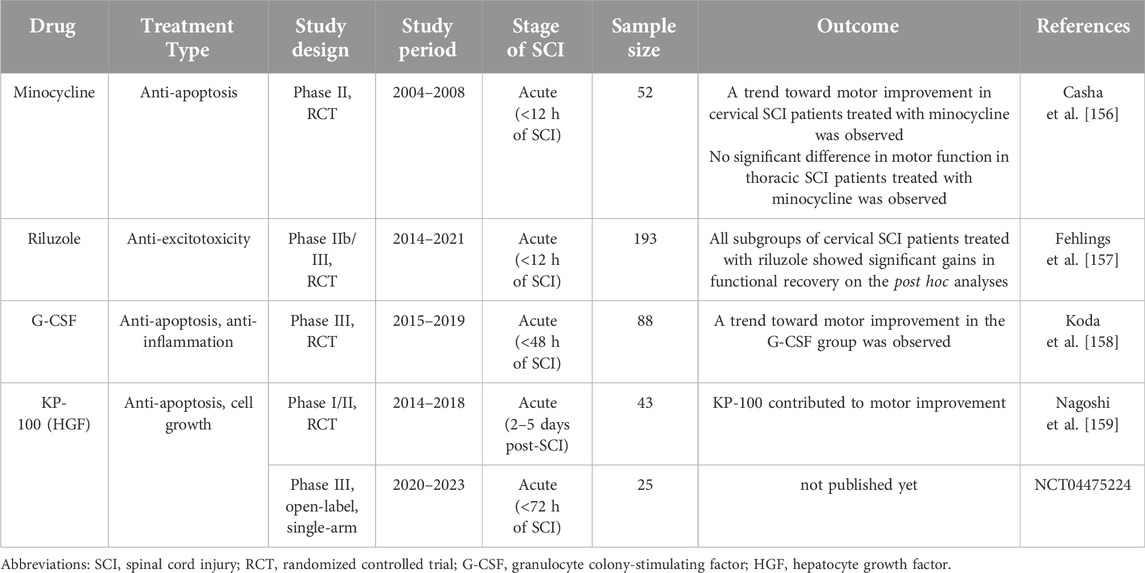
Table 1. Summary of leading completed and ongoing clinical trials regarding neuroprotective therapies for spinal cord injury.
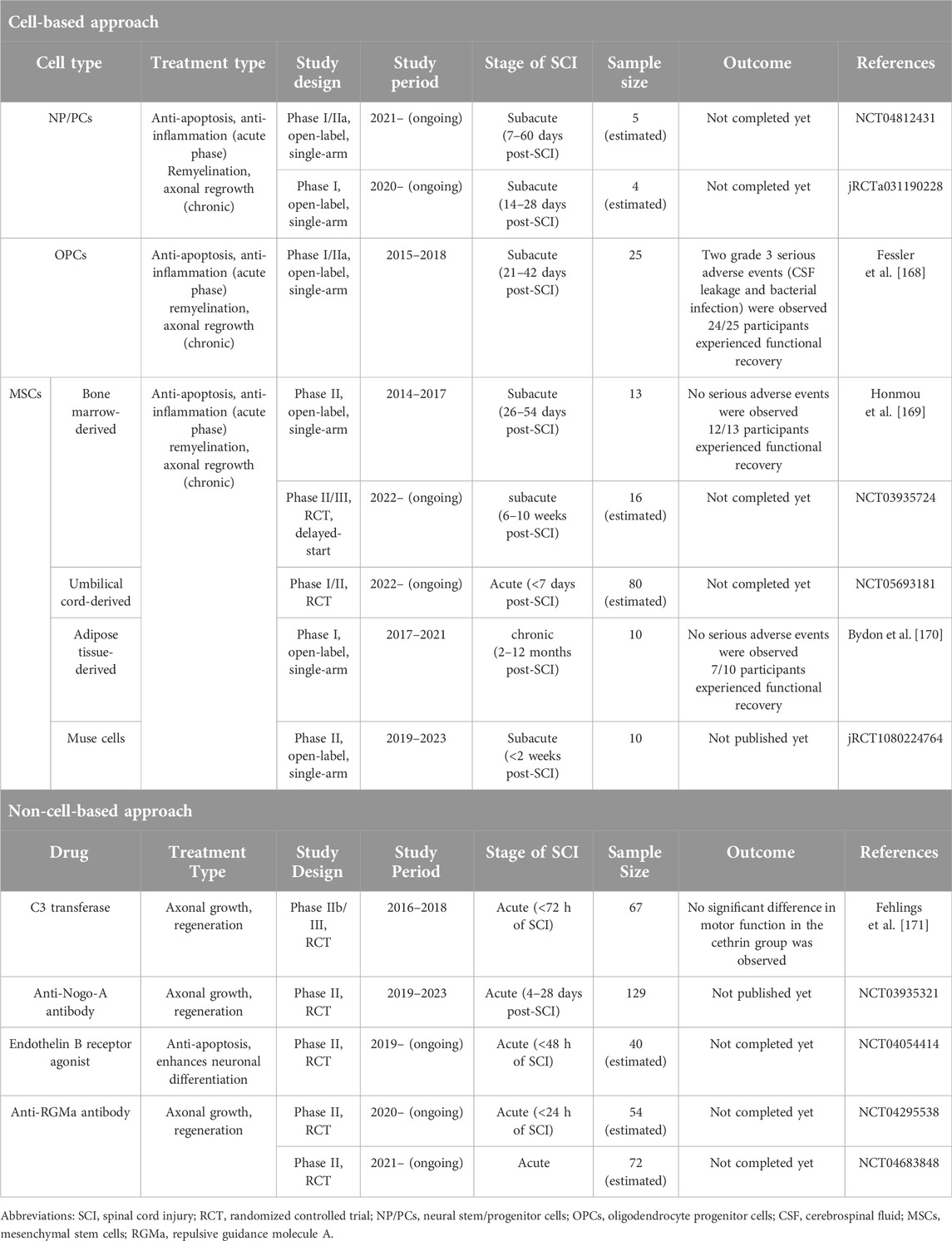
Table 2. Summary of leading completed and ongoing clinical trials regarding regenerative therapies for spinal cord injury.
Neuroprotective therapy
Minocycline
Minocycline is a tetracycline antibiotic used clinically as an antimicrobial agent. It also exhibits anti-apoptotic characteristics through the inhibition of caspase-1 and –3 [160, 161]. A phase II RCT (NCT00559494) conducted from 2004 to 2008 demonstrated safety and a trend toward motor function improvement, as measured by the ASIA motor score, in cervical SCI patients treated with minocycline (14 points; 95% CI: 0–28; p = 0.05) [156]. Based on these promising results, a phase III RCT, Minocycline in Acute Spinal Cord Injury (MACS) (NCT01828203), was initiated in 2013. However, this trial was discontinued, and its results have not yet been published.
Granulocyte colony stimulating factor (G-CSF)
G-CSF, known as a growth factor for hematopoietic cells, has also demonstrated neuroprotective characteristics for SCI through angiogenesis, inflammation suppression, and apoptosis inhibition in preclinical research [162–164]. An open-label phase I/IIa trial of G-CSF for acute SCI conducted from 2008 to 2010 revealed no severe adverse events related to G-CSF administration [165]. Another open-label, non-randomized controlled phase II trial carried out between 2009 and 2011 by the same group found a significantly greater improvement in the ASIA motor score in the G-CSF group compared to the control group [166]. Encouraged by these promising results, a phase III RCT, the G-CSF mediated spinal cord injury recovery induction trial (G-SPIRIT) (UMIN000018752), was initiated in 2015. Although this trial reported no significant differences in the primary efficacy endpoint, measured by changes in the ASIA motor score at 3 months post-intervention between the G-CSF and control groups, those at 6 and 12 months showed a trend towards better improvement in the G-CSF group [158].
Hepatocyte growth factor
Hepatocyte growth factor (HGF) is secreted by mesenchymal stem cells and regulates cell growth and cell motility by activating a tyrosine kinase signaling cascade through binding to the c-Met receptor. In preclinical research using primate models, HGF enhanced motor neuron survival and reduced cavitation at the injured site [167]. A phase I/II RCT using recombinant human HGF (KP-100IT) for acute SCI (NCT02193334) was conducted starting in 2014. It demonstrated safety and suggested improvements in motor function as evaluated by the ASIA motor score [159]. Based on these results, an open-label, single-arm phase III trial (NCT04475224) was conducted. However, it appears not to have achieved its primary efficacy endpoints, potentially influenced by variations in patients’ baselines due to the COVID-19 pandemic. Detailed results, including any post hoc analyses, are to be published in the near future.
Regenerative therapy (Table 2)
Cell-based therapy
Cell-based therapies are a promising strategy for the treatment of SCI, offering a variety of therapeutic mechanisms. Supported by substantial pre-clinical evidence, numerous clinical trials have been conducted.
Neural stem/progenitor cells (NS/PCs)
NS/PCs, capable of self-renewal and differentiating into neurons and glial cells, have been utilized in several clinical trials. Two clinical trials include an open-label phase I/II trial for chronic thoracic SCI (NCT01321333) and a single-blinded phase II RCT for chronic cervical SCI (NCT02163876), where human fetal brain-derived NS/PCs were transplanted into the spinal cord around the epicenter. These trials indicated no serious adverse events related to the intramedullary injection or additional spinal cord damage; however, they failed to demonstrate the efficacy anticipated by the sponsor [172–174]. Another open-label, single-arm phase I trial with the intramedullary transplantation of human spinal cord-derived NS/PCs for chronic thoracic SCI (NCT01772810) began in 2014, with 5-year follow-up results recently reported. According to this report, no serious adverse events were directly attributed to cell transplantation [175]. Currently, there are ongoing clinical trials using human embryonic stem cell (ESC)-derived NS/PCs and human induced pluripotent stem cell (iPSC)-derived NS/PCs. An open-label, single-arm phase I/IIa trial with human ESC-derived NS/PCs (NCT04812431) is targeting subacute cervical SCI and is estimated to be completed by 2028. Another open-label, single-arm phase I trial using human iPSC-derived NS/PCs (UMIN000035074, jRCTa031190228) is targeting subacute cervical or thoracic SCI [176]. This trial is expected to be completed by 2024 and aims to address ethical concerns associated with deriving NS/PCs from human ESC or fetuses.
Oligodendrocyte progenitor cells (OPCs)
OPCs are also self-renewing, multipotent cells that preferentially differentiate into oligodendrocytes, as opposed to NS/PCs. Preclinical studies have demonstrated their capability to secrete neurotrophic factors, suppress inflammation, remyelinate axons, and spare tissues [177–180]. An open-label, single-arm phase I trial (NCT01217008) was conducted from 2010 to 2013, involving the direct transplantation of OPCs into the injured epicenter in patients with subacute thoracic SCI. This study confirmed their safety for up to 10 years post-SCI [181]. Based on this safety profile, an open-label, single-arm phase I/IIa trial for subacute cervical SCI (NCT02302157) took place from 2015 to 2018. Results from this trial indicated not only the safety of OPCs but also functional improvements as assessed by the International Standards for Neurological Classification of Spinal Cord Injury examination at 1-year post-SCI [168]. Consequently, a phase III trial to confirm their efficacy is now warranted.
Schwann cells (SCs)
SCs have shown the ability to promote remyelination, improve axonal sparing, and reduce the inflammatory response in preclinical studies [182–184]. Two open-label, single-arm phase I trials of SCs for SCI have been conducted by the Miami Project to Cure Paralysis. The first one (NCT01739023) was performed between 2012 and 2015 and enrolled six patients with subacute thoracic SCI [185]. Another trial (NCT02354625) was conducted from 2015 to 2019 and enrolled six patients with chronic (more than 1 year) thoracic SCI [186]. In both trials, SCs were harvested from the sural nerve of the participants, and autologously transplanted into the epicenter of SCI, with no serious adverse events being reported. However, no evidence of its efficacy has been reported to date.
Mesenchymal stem cells (MSCs)
MSCs can exert immunomodulatory, anti-inflammatory, neuroprotective, and angiogenic effects by secreting numerous trophic factors [187], This secretion improves the local environment of the injured spinal cord. Due to their ability to migrate to the injured lesion [188], MSCs can be transplanted directly into the injured site or via intravenous injection, offering a less invasive option for patients.
MSCs can be derived from multiple sources, including bone marrow (BM), umbilical cord (UC), adipose tissue (AD), Wharton’s jelly, and amnion. Their efficacy has been demonstrated in several preclinical studies [189–193]. Based on these sources, numerous clinical trials have been conducted. For instance, autologous BM-MSCs were intravenously injected in an open-label, single-arm phase II trial for subacute cervical SCI (JMA-IIA00154). This trial reported no serious adverse events related to the cell injection and showed neurological improvement [169]. Based on the results, the MSC product (Stemirac®) has been approved through the conditional early approval program in Japan, although further evaluations are required to determine its efficacy. Additionally, a double-blinded, placebo-controlled, and delayed-start phase II/III trial, Stem Cells in Spinal Cord Injury (SCI2) (NCT03935724), began in 2022. In this trial, patients with subacute cervical and thoracic SCI are intrathecally injected with the BM-MSC product (Neuro-Cells). The trial is expected to be completed by 2024.
Regarding UC-MSCs, allogeneic UC-MSCs were administered intravenously in an open-label phase I/IIa RCT for acute SCI (NCT04331405). This trial showed only two mild adverse events (transitory mild hyperthermia after cell infusion) and most patients experienced neurological improvement, although the results from the phase IIa trial are still pending [194]. Currently, the same group is conducting a single-blinded phase I/II RCT for acute SCI, Systemic Umbilical Cord Blood Administration in Patients with Acute Severe Contusion Spinal Cord Injury II (SUBSCI II) (NCT05693181), which began in 2022 and is estimated to be completed by 2025.
As for AD-MSCs, an open-label, single-arm phase I trial, the Adipose Stem Cells for Traumatic Spinal Cord Injury (CELLTOP) (NCT03308565), was conducted at the Mayo Clinic from 2017 to 2021. In this trial, participants with subacute and chronic SCI intrathecally received AD-MSCs. Recent results indicated no serious adverse events, with 7 out of 10 participants showing improvement in AIS grade post-injection [170]. Another phase I/II RCT for acute thoracic SCI (NCT02917291) involved transplanting allogenic AD-MSCs (FAB117-HC) into the injured spinal cord. This trial was expected to be completed by 2023, though its current status is unknown.
Multilineage-differentiating stress-enduring (Muse) cells, identified as stress-tolerant pluripotent stem cells within MSCs, are promising for SCI therapy [195]. Muse cells recognize injured sites with sphingosine-1-phosphate (S1P) via the S1P receptor 2 and migrate accordingly. A preclinical study showed that they reduced cystic cavities and preserved axons [196]. An open-label single-arm phase II trial using Muse cells (CL2020) for acute/subacute cervical SCI (jRCT1080224764) was conducted in Japan. The trial was completed in 2023, and the results are expected to be reported in the near future.
C3 transferase
C3 transferase inhibits Rho signaling, consequently promoting axonal growth and regeneration [197, 198]. An open-label, single-arm, phase I/IIa trial (NCT00500812) was conducted from 2005 to 2009, and Cethrin, a recombinant C3 transferase, was applied to the surface of the dura mater overlying the injured lesion during decompressive surgery [199]. No serious adverse events were reported, and then, a phase IIb/III RCT, SPinal Cord Injury Rho INhibition InvestiGation (SPRING), was conducted from 2016 to 2018 (NCT02669849). Unfortunately, this trial was terminated because the interim efficacy results, evaluated by the upper-extremity motor score, did not show a significant difference between the C3 transferase group and the placebo [171].
Anti-Nogo-A antibody
Nogo-A is one of the myelin-associated proteins that inhibit neuronal growth by activating the Rho/ROCK pathway upon binding to the Nogo receptor [200]. Therefore, the anti-Nogo-A antibody has the potential to improve axonal regrowth by mediating Rho/ROCK signaling, as demonstrated in preclinical research using primate models [201]. An open-label phase I trial utilizing recombinant anti-Nogo-A antibody (ATI355) for acute traumatic SCI (NCT00406016) was performed from 2006 to 2011 and found no drug-related serious adverse events [202]. A phase II RCT trial using anti-Nogo-A antibody (NG-101) for acute cervical SCI, Nogo Inhibition in Spinal Cord Injury (NISCI) (NCT03935321), was initiated in 2019 and recently completed in 2023. In this trial, the anti-Nogo-A antibody treatment did not show a statistically significant benefit for the primary efficacy endpoint. The detailed results, including post hoc analysis, have not yet been published. Similarly, a phase I/II trial using a soluble Nogo-Receptor-Fc decoy (AXER-204) for chronic cervical SCI, ReNetX Safety Efficacy and Tolerability of AXER-204 for Chronic SCI (RESET) (NCT03989440), was conducted from 2019 to 2022. It demonstrated safety; however, no significant differences were observed for secondary efficacy endpoints between the AXER-204 group and the placebo group [203].
Endothelin B receptor agonist
Sovateltide, also known as IRL-1620 or PMZ-1620, is an endothelin B receptor agonist that has enhanced neuronal differentiation and reduced apoptosis in animal models of cerebral infarction [204, 205]. Following the promising results of a RCT with Sovateltide for acute cerebral ischemic stroke [206], a phase II RCT of PMZ-1620 for acute SCI (NCT04054414) was initiated in 2019. The trial is currently ongoing and is estimated to be completed in 2024.
Anti-repulsive guidance molecule A (RGMa) antibody
RGMa is a protein that activates the RhoA-Rho kinase pathway and consequently inhibits axonal regeneration [207]. In preclinical research using a primate model of SCI, an RGMa antibody facilitated the recovery of manual dexterity by enhancing the penetration of corticospinal tract fibers into laminae VII and IX [208]. To date, a few clinical trials are ongoing. Phase II RCT using a human anti-RGMa monoclonal antibody, known as Elezanumab (ABT-555), for acute SCI (NCT04295538) was initiated in 2020 and is estimated to be completed in 2026. Additionally, the expanded access program for Elezanumab has been approved (NCT04278235). Another phase II RCT using MT-3921 for acute cervical SCI (NCT04683848) was started in 2021 and is estimated to be completed in 2025.
Others
CSF drainage
As SCPP is determined by the difference between MAP and intraspinal pressure, CSF drainage through a lumbar intrathecal catheter placed into the subarachnoid space is another method to maintain spinal cord blood flow. This procedure is commonly utilized for patients undergoing thoracoabdominal aortic aneurysm repair surgery, which potentially has a risk of spinal cord ischemia due to hypoperfusion from the important segmental artery connected to the anterior spinal artery [209]. A phase I clinical trial for acute SCI (NCT00135278) that commenced in 2006 indicated that CSF drainage did not result in any significant adverse events. However, it failed to demonstrate a significant difference in the ASIA motor score, likely due to the small sample size [210]. Following this trial, a phase II RCT was conducted from 2015 to 2019 (NCT02495545) to compare outcomes between MAP maintenance with CSF drainage and MAP maintenance alone. The results of this trial have not yet been published.
Therapeutic hypothermia
Therapeutic hypothermia is used in various medical scenarios to minimize secondary damage to the central nervous system. For instance, the 2020 American Heart Association Guidelines for cardiopulmonary resuscitation recommends that the target temperature for patients who achieve return of spontaneous circulation should be maintained between 32°C and 36°C for at least 24 h [211].
Therapeutic hypothermia is also regarded as a neuroprotective strategy for acute SCI. Several preclinical and clinical studies have suggested that this procedure might improve behavioral outcomes [212, 213]. A RCT comparing systemic hypothermia with standard treatment (NCT02991690) was initiated in 2017 and is expected to be completed by 2024. According to the interim report published in 2022 [214], preliminary data indicated that modest systemic hypothermia (33°C for 48 h) following acute SCI was not associated with an increased risk of complications. The results are anticipated to clarify the efficacy of therapeutic hypothermia.
Examples of therapies at the advanced stages of translation
Riluzole
Riluzole, a benzothiazole approved by the FDA for the treatment of amyotrophic lateral sclerosis, acts as a neuroprotective agent. It blocks sodium channels and reduces glutamate-associated excitotoxicity by decreasing glutamate release from the presynaptic terminal, preventing glutamate receptor hypofunction, and stimulating glutamate uptake [215]. Following SCI, voltage-sensitive sodium channels are constitutively activated, leading to increased intracellular sodium concentration, cellular swelling, and intracellular acidosis [216]. Additionally, the increase in intracellular sodium facilitates the influx of calcium ions through the Na+/Ca2+ exchanger, resulting in the extracellular release of excess glutamate and localized cell death. Riluzole is well-suited to inhibit these processes involved in secondary injury.
Based on promising results from several pre-clinical studies supporting the effectiveness of riluzole for acute SCI [217, 218], a phase I clinical trial for demonstrating the safety of riluzole in acute SCI (NCT00876889) was conducted between 2010 and 2012. This trial demonstrated that there were no serious adverse events associated with riluzole [219]. Additionally, patients with cervical SCI treated with riluzole had a significantly higher mean ASIA motor score at 90 days post-SCI compared to matched patients in the North American Clinical Trials Network SCI Registry (31.2 points vs. 15.7 points; p = 0.021). These encouraging results led to a double-blind phase IIb/III RCT, the Riluzole in Acute Spinal Cord Injury Study (RISCIS) (NCT01597518), initiated in 2014. Originally planned to enroll 351 patients, the trial was terminated in 2021 with 193 participants due to the COVID-19 pandemic. The primary efficacy outcome of the Upper Extremity Motor score at 180 days post-SCI showed no significant difference between the riluzole and control groups, likely due to insufficient power [157]. However, post hoc analysis revealed some hopeful results; for instance, the Upper Extremity and Total Motor score in the AIS C population treated with riluzole at 180 days post-SCI were significantly better than those without, according to multivariate linear regression models. Although this trial could not definitively determine the efficacy of riluzole, Fehlings et al. concluded that riluzole could be considered as one of therapeutic options in the clinical settings, given the lack of alternative pharmacological treatments for severe SCI. Currently improved techniques for trial design and handling the heterogeneity of patients will enhance future results.
Additionally, a phase III RCT for chronic cervical SCI (NCT01257828) was also conducted between 2012 and 2017. This trial did not show significant difference between the riluzole and control groups in the primary efficacy endpoint measured by the change in the modified Japanese Orthopaedic Association score at 6 months post-intervention [220]; however, the latest secondary analyses using a global statistical test showed a significant functional improvement at 1-year post-intervention in the riluzole group compared to the control group (in press). Riluzole remains a promising pharmaceutical treatment for SCI.
Neuromodulation and stimulation
The main goal of rehabilitation strategies after SCI is to enhance functional recovery [221]. One possible way to achieve this goal is to strengthen the efficacy of the residual neuronal pathways [222, 223]. Electrical and magnetic neural stimulation induces significant and long-lasting neuroplastic effects that involve neuroplasticity markers [222, 223].
Transcranial magnetic stimulation
Non-invasive repetitive transcranial magnetic stimulation (rTMS) has been applied to target sensory and motor function impairments, spasticity, and neuropathic pain [222]. The influence of rTMS in patients with SCI may confirm the hypothesis about the significance of the propriospinal system and other residual efferent pathways in the recovery of motor control [224]. Moreover, rTMS targeted at the motor cortex has suggested therapeutic potential in alleviating chronic neuropathic pain [225–228], indicating its beneficial effects in evaluating and enhancing motor function in SCI patients [224, 229] (Table 3).
Electrical stimulation
Electrical stimulation can accelerate axonal growth and myelination [240], stimulate neurons to discharge bioelectric signals to strengthen muscle contraction, and reconnect the neural network of the spinal cord [241]. Therefore, the baseline excitability of neural circuits is regulated by electrical stimulation, leading to action potentials within and between neural circuits by adjusting excitability to a precise level [242]. Electrical stimulation also enhances neurotransmitter release capacity via recruiting local neurotransmitters across synaptic sites by stimulating the amount of neurostimulation of afferent nerve fibers [242]. Moreover, epidural electrical stimulation leads to greater recovery of motor output after a severe SCI, and with intensive training and electrical stimulation, recovery of walking, standing, and trunk mobility can occur years after SCI [235]. Within a single day, activity-specific stimulation programs have enabled standing, walking, cycling, swimming, and control of trunk movements [236]. In addition, Transcutaneous Spinal Cord Stimulation (SCS) shows promise in reducing spasticity [230]. The application of this non-invasive spinal cord electrical stimulation technique is safe and effective for improving hand and arm function in individuals with cervical SCI (Table 3) [231].
Electrical stimulation and neuromodulation strategies show promise for enhancing motor function in SCI patients. Despite their potential, the use of electrical and magnetic stimulation in SCI rehabilitation faces several considerations and limitations, necessitating significant validation through clinical trials. Additionally, methodological variability across studies complicates the interpretation of outcomes. Standardization of stimulation parameters, patient selection criteria, and outcome measures are essential to ease meaningful comparisons and robust conclusions regarding stimulation effectiveness in SCI rehabilitation.
Neurorehabilitation
Various types of motor training such as bicycling, swimming, and locomotor training decrease the inflammatory response, increase neurotrophins, and may strengthen spared functions and guide spinal reorganization [83]. Exercise has been shown to preserve muscle mass [243], restore motor and sensory function [232, 244, 245], induce synaptic plasticity [246], increase the concentration of neurotrophic factors in spinal and muscle tissue [247, 248], and reduce inflammation around the injured site [245] (Table 3).
Studies investigating the timing of exercise post-injury suggest it may yield advantageous or adverse consequences on the recovery outcomes [249–251]. Despite numerous studies, several questions remain unanswered regarding therapeutic tools, such as optimal rehabilitation timing, the most suitable intensity, duration, and frequency, as well as the best use of task-specific training for recovery of various functional modalities.
Robot rehabilitation and brain–computer interfaces
In recent years, neural interfaces such as Brain-computer interfaces (BCIs) have used physiological brain activity to control external devices, thereby enabling severely disabled patients to interact with the outside environment [252]. Invasive and non-invasive BCI approaches have been used to promote neural control of a robotic arm [253] for patients with severe paralysis. This system has the potential to provide a new way of controlling their wheelchair [238, 239, 254].
All approaches to adaptive technology for patients with SCI have aimed to provide patients with control of their paralyzed limbs [233, 234, 237]. However, the possibility that similar systems could bring neuroplastic alterations that contribute to functional rehabilitation remains unclear. The technologies in combination with current pharmacological and neurostimulation approaches may offer a crucial pathway to motor recovery following SCI [255, 256].
Ethical considerations
While the medical concerns of SCI such as neuropathic pain and spasticity have received significant attention, a smaller number of works have focused on ethical issues related to treatment and research in SCI, such as voluntary consent, patient welfare, transparency, medical decision-making, and the patient-physician relationship [257, 258]. The perspectives, priorities, and experiences of individuals living with SCI are influenced by social, environmental, clinical, and injury-associated aspects [259]. In addition, living with SCI is aggravated by a fair treatment concern and sociocultural factors at a systems level, such as a lack of accessible support, information, and rehabilitation as well as healthcare services, which create hindrance to reunification of research and clinical studies [260].
SCI research raises crucial ethical questions concerning participant welfare and the use of funding [257–260]. The approach of a consistent clinical trial plays a very important role in the reliability of the research, especially the inclusion and exclusion criteria, ethical issues, treatment uniformity, and informed consent [258, 259]. The inclusion and exclusion criteria to control the consistency of the trial must be developed based on the specific research content [259, 261]. Informed consent for clinical trials as well as the standardization of the operation procedures and rehabilitation treatment is necessary [261].
However, within SCI research, the concept of data sharing, meta-analysis, and the application of new statistical techniques such as recursive partitioning and the global statistical test show promise. A notable challenge is that publications focusing on preclinical research often present only a fraction of the generated data [262]. This limitation may be attributed, in part, to the constraints imposed by journals on word counts as well as the number of figures and tables allowed. Nonetheless, in recent years, various stakeholders within the SCI research community have actively advocated for publication standards and promoted the sharing of experimental data. Initiatives like The Open Data Commons for Spinal Cord Injury (ODC-SCI.org) have emerged, facilitating data sharing and enabling pooled data-driven discoveries while appropriately acknowledging the contributors of valuable SCI data [261].
Research involving individuals with SCI must prioritize patient well-being and minimize any potential harm or discomfort related to experimental procedures [263–265]. In addition, clear communication about the risk factors and potential benefits of research is essential; therefore, SCI patients should have access to information about the research process and its implications. Moreover, transparency regarding funding, prioritizing research subjects with the greatest potential to improve quality of life and develop new treatments, adhering to established standards of scientific integrity, accurately reporting findings, avoiding data manipulation, and community engagement to promote ethical conduct are key factors in clinical trial studies [263–265].
The burden of SCI and inequality in the international context
The challenges faced by individuals with SCI across different countries and regions around the world include issues such as access to healthcare, rehabilitation services, and supporting devices. In many parts of the world, there is a lack of resources and support systems for people living with SCI, leading to increased vulnerability and inequality. Understanding and addressing these issues on a global scale is crucial for improving the quality of life and outcomes for individuals with SCI worldwide [266].
Unaddressed healthcare needs are significant for SCI patients, where people in low-income groups tend to be more affected. Among the barriers to meeting healthcare needs are healthcare cost, transportation, and service availability [266]. To improve the situation, a combination of measures from the health and social systems are required. Improving access to healthcare to ensure individuals with SCI have access to affordable and appropriate assistive technologies, specialized medical care, and rehabilitation services. This can improve functional outcomes and quality of life for all SCI patients regardless of their socioeconomic status. Providing equal access to education and employment opportunities for individuals with SCI and promoting public awareness to reduce stigma contributes to creating a more equitable and inclusive world for individuals living with SCI globally [266, 267].
Future directions: combinatorial therapies
As each therapy seeks to target aspects of SCI, combinatorial approaches are now emerging to match the disease timeline and improve functional outcomes. Exemplifying the notion of combinatorial approaches to SCI can be done with the strategies currently available. Rehabilitative as well as FES (functional electrical stimulation) therapies rely on the presence of remaining neurons and parenchyma for the establishment of new synapses and subsequent functional recovery. Complementing rehabilitation and FES are early surgical intervention and stem cell therapies. Early surgical intervention prevents further secondary injury through reperfusion of the injured spinal cord, increasing the net preserved CNS tissue for synapse recruitment as well as reducing inflammation and scarring hostile to recovery. Similarly, stem cell therapy can be used for the replacement of lost cells in the CNS as well as creating a microenvironment conducive to repair through reduced inflammation. This potentially gives greater neuronal and synapse recruitment for better functional outcomes and impact from rehabilitation and FES.
Pharmaceutical options are currently limited but follow a similar trajectory, with each drug potentially playing a different role dependent on which aspect of SCI injury is targeted. The growing movement towards personalized medicine is highly applicable to the heterogeneous nature of SCI. There is a great degree of variability in effectiveness and perceived outcomes between treatments across patients. This has been highlighted particularly well by patient perceptions on the effectiveness of different treatments for chronic pain. Preferences have been shown for different opioid medications, diazepam, rehabilitative exercise, as well as massage – all to differing degrees [268, 269].
Conclusion
There have been remarkable advancements in medical, surgical, and rehabilitative treatments for SCI. However, despite these advances, opportunities exist to develop reparative and regenerative approaches to enhance outcomes. Although it remains true that the outcomes in SCI improve in a very incremental fashion due to the complex nature of SCI pathophysiology, the advancements in techniques and strategies are impressively thorough and creative. By incorporating patient-centric approaches, insight into individual differences can guide current and emergent treatment as well as provide better autonomy to patients. Adapting each regenerative approach into a cohesive strategy in concert remains a difficult task yet even modest improvements in sensory and motor function returning to patients can be both meaningful and motivating in the face of a highly debilitating disease.
Author contributions
AA: Conceptualization, Data curation, Investigation, Methodology, Visualization, Writing–original draft, Writing–review and editing. MGF: Conceptualization, Investigation, Methodology, Project administration, Resources, Supervision, Writing–original draft, Writing–review and editing. OH: Conceptualization, Data curation, Investigation, Methodology, Visualization, Writing–original draft, Writing–review and editing. ST: Conceptualization, Data curation, Investigation, Methodology, Visualization, Writing–original draft, Writing–review and editing.
Acknowledgments
MF would like to acknowledge support from the Robert Campeau Family Foundation/Dr. C. H. Tator Chair in Brain and Spinal Cord Research at UHN.
Conflict of interest
The authors declared no potential conflicts of interest with respect to the research, authorship, and/or publication of this article.
References
1. Ahuja, CS, Wilson, JR, Nori, S, Kotter, MRN, Druschel, C, Curt, A, et al. Traumatic spinal cord injury. Nat Rev Dis Primers (2017) 3(1):17018–21. doi:10.1038/nrdp.2017.18
2. National Spinal Cord Injury Statistical Center. Spinal cord injury facts and figures at a glance. J Spinal Cord Med. (2014) 37(1):117–8. doi:10.1179/1079026813Z.000000000249
3. Khaing, ZZ, Chen, JY, Safarians, G, Ezubeik, S, Pedroncelli, N, Duquette, RD, et al. Clinical trials targeting secondary damage after traumatic spinal cord injury. Int J Mol Sci (2023) 24(4):3824. doi:10.3390/ijms24043824
4. Singh, A, Tetreault, L, Kalsi-Ryan, S, Nouri, A, and Fehlings, MG. Global prevalence and incidence of traumatic spinal cord injury. Clin Epidemiol (2014) 6:309–31. doi:10.2147/clep.s68889
5. Chiu, WT, Lin, HC, Lam, C, Chu, SF, Chiang, YH, and Tsai, SH. Review paper: epidemiology of traumatic spinal cord injury: comparisons between developed and developing countries. Asia Pac J Public Health (2010) 22(1):9–18. doi:10.1177/1010539509355470
6. Chen, Y, He, Y, and DeVivo, MJ. Changing demographics and injury profile of new traumatic spinal cord injuries in the United States, 1972–2014. Arch Phys Med Rehabil (2016) 97(10):1610–9. doi:10.1016/j.apmr.2016.03.017
7. DeVivo, MJ. Epidemiology of traumatic spinal cord injury: trends and future implications. Spinal Cord (2012) 50(5):365–72. doi:10.1038/sc.2011.178
8. Chan, WM, Mohammed, Y, Lee, I, and Pearse, DD. Effect of gender on recovery after spinal cord injury. Transl Stroke Res (2013) 4(4):447–61. doi:10.1007/s12975-012-0249-7
9. Scivoletto, G, Morganti, B, Ditunno, P, Ditunno, JF, and Molinari, M. Effects on age on spinal cord lesion patients’ rehabilitation. Spinal Cord (2003) 41(8):457–64. doi:10.1038/sj.sc.3101489
10. Pickett, GE, Campos-Benitez, M, Keller, JL, and Duggal, N. Epidemiology of traumatic spinal cord injury in Canada. Spine (Phila Pa 1976) (2006) 31(7):799–805. doi:10.1097/01.brs.0000207258.80129.03
11. O’Connor, RJ, and Murray, PC. Review of spinal cord injuries in Ireland. Spinal Cord (2006) 44(7):445–8. doi:10.1038/sj.sc.3101856
12. Karacan, İ, Koyuncu, H, Pekel, Ö, Sümbüloğlu, G, Kırnap, M, Dursun, H, et al. Traumatic spinal cord injuries in Turkey: a nation-wide epidemiological study. Spinal Cord (2000) 38(11):697–701. doi:10.1038/sj.sc.3101064
13. Miyakoshi, N, Suda, K, Kudo, D, Sakai, H, Nakagawa, Y, Mikami, Y, et al. A nationwide survey on the incidence and characteristics of traumatic spinal cord injury in Japan in 2018. Spinal Cord (2021) 59(6):626–34. doi:10.1038/s41393-020-00533-0
14. Rahimi-Movaghar, V, Sayyah, MK, Akbari, H, Khorramirouz, R, Rasouli, MR, Moradi-Lakeh, M, et al. Epidemiology of traumatic spinal cord injury in developing countries: a systematic review. Neuroepidemiology (2013) 41(2):65–85. doi:10.1159/000350710
15. Sekhon, LHS, and Fehlings, MG. Epidemiology, demographics, and pathophysiology of acute spinal cord injury. Spine (2001) 26(24S):S2–S12. doi:10.1097/00007632-200112151-00002
16. Krause, JS, Sternberg, M, Lottes, S, and Maides, J. Mortality after spinal cord injury: an 11-year prospective study. Arch Phys Med Rehabil (1997) 78(8):815–21. doi:10.1016/s0003-9993(97)90193-3
17. Furlan, JC, and Fehlings, MG. The impact of age on mortality, impairment, and disability among adults with acute traumatic spinal cord injury. J Neurotrauma (2009) 26(10):1707–17. doi:10.1089/neu.2009.0888
18. Furlan, JC, Kattail, D, and Fehlings, MG. The impact of Co-morbidities on age-related differences in mortality after acute traumatic spinal cord injury. J Neurotrauma (2009) 26(8):1361–7. doi:10.1089/neu.2008.0764
19. Le Fort, M, Lefèvre, C, Kieny, P, Perrouin-Verbe, B, and Ravaud, JF. The functioning of social support in long-term prevention after spinal cord injury. A qualitative study. Ann Phys Rehabil Med (2021) 64(4):101454. doi:10.1016/j.rehab.2020.10.007
20. Espagnacq, MF, Albert, T, Boyer, FC, Brouard, N, Delcey, M, Désert, JF, et al. Predictive factors of long-term mortality of persons with tetraplegic spinal cord injury: an 11-year French prospective study. Spinal Cord (2011) 49(6):728–35. doi:10.1038/sc.2010.189
21. Zavvarian, MM, Hong, J, and Fehlings, MG. The functional role of spinal interneurons following traumatic spinal cord injury. Front Cel Neurosci (2020) 14:127. doi:10.3389/fncel.2020.00127
22. Punjani, N, Deska-Gauthier, D, Hachem, LD, Abramian, M, and Fehlings, MG. Neuroplasticity and regeneration after spinal cord injury. North Am Spine Soc J (Nassj) (2023) 15:100235. doi:10.1016/j.xnsj.2023.100235
23. Hachem, LD, and Fehlings, MG. Pathophysiology of spinal cord injury. Neurosurg Clin North America (2021) 32(3):305–13. doi:10.1016/j.nec.2021.03.002
24. Hachem, LD, Hong, J, Velumian, A, Mothe, AJ, Tator, CH, and Fehlings, MG. Excitotoxic glutamate levels drive spinal cord ependymal stem cell proliferation and fate specification through CP-AMPAR signaling. Stem Cel Rep (2023) 18(3):672–87. doi:10.1016/j.stemcr.2023.01.005
25. Ankeny, DP, and Popovich, PG. Mechanisms and implications of adaptive immune responses after traumatic spinal cord injury. Neuroscience (2009) 158(3):1112–21. doi:10.1016/j.neuroscience.2008.07.001
26. Aubé, B, Lévesque, SA, Paré, A, Chamma, É, Kébir, H, Gorina, R, et al. Neutrophils mediate blood–spinal cord barrier disruption in demyelinating neuroinflammatory diseases. J Immunol (2014) 193(5):2438–54. doi:10.4049/jimmunol.1400401
27. Acarin, L, González, B, and Castellano, B. Neuronal, astroglial and microglial cytokine expression after an excitotoxic lesion in the immature rat brain. Eur J Neurosci (2000) 12(10):3505–20. doi:10.1046/j.1460-9568.2000.00226.x
28. Dumont, RJ, Okonkwo, DO, Verma, S, Hurlbert, RJ, Boulos, PT, Ellegala, DB, et al. Acute spinal cord injury, Part I: pathophysiologic mechanisms. Clin Neuropharmacology (2001) 24(5):254–64. doi:10.1097/00002826-200109000-00002
29. Rodriguez-Jimenez, FJ, Jendelova, P, and Erceg, S. The activation of dormant ependymal cells following spinal cord injury. Stem Cel Res and Ther (2023) 14(1):175. doi:10.1186/s13287-023-03395-4
30. Ren, Y, Ao, Y, O’Shea, TM, Burda, JE, Bernstein, AM, Brumm, AJ, et al. Ependymal cell contribution to scar formation after spinal cord injury is minimal, local and dependent on direct ependymal injury. Sci Rep (2017) 7(1):41122. doi:10.1038/srep41122
31. Bellver-Landete, V, Bretheau, F, Mailhot, B, Vallières, N, Lessard, M, Janelle, ME, et al. Microglia are an essential component of the neuroprotective scar that forms after spinal cord injury. Nat Commun (2019) 10(1):518. doi:10.1038/s41467-019-08446-0
32. Maikos, JT, and Shreiber, DI. Immediate damage to the blood-spinal cord barrier due to mechanical trauma. J Neurotrauma (2007) 24(3):492–507. doi:10.1089/neu.2006.0149
33. Jin, LY, Li, J, Wang, KF, Xia, WW, Zhu, ZQ, Wang, CR, et al. Blood-spinal cord barrier in spinal cord injury: a review. J Neurotrauma (2021) 38(9):1203–24. doi:10.1089/neu.2020.7413
34. Noble, LJ, and Wrathall, JR. Distribution and time course of protein extravasation in the rat spinal cord after contusive injury. Brain Res (1989) 482(1):57–66. doi:10.1016/0006-8993(89)90542-8
35. Popovich, PG, Horner, PJ, Mullin, BB, and Stokes, BT. A quantitative spatial analysis of the blood–spinal cord barrier. Exp Neurol (1996) 142(2):258–75. doi:10.1006/exnr.1996.0196
36. Noble, LJ, and Wrathall, JR. The blood-spinal cord barrier after injury: pattern of vascular events proximal and distal to a transection in the rat. Brain Res (1987) 424(1):177–88. doi:10.1016/0006-8993(87)91208-x
37. Noble, LJ, and Wrathall, JR. Blood-spinal cord barrier disruption proximal to a spinal cord transection in the rat: time course and pathways associated with protein leakage. Exp Neurol (1988) 99(3):567–78. doi:10.1016/0014-4886(88)90173-2
38. Demediuk, P, Saunders, RD, Anderson, DK, Means, ED, and Horrocks, LA. Early membrane lipid changes in laminectomized and traumatized cat spinal cord. Neurochem Pathol (1987) 7(1):79–89. doi:10.1007/bf02834293
39. Griffiths, IR, and McCulloch, MC. Nerve fibres in spinal cord impact injuries. J Neurol Sci (1983) 58(3):335–49. doi:10.1016/0022-510x(83)90093-x
40. Sharma, H. Pathophysiology of blood-spinal cord barrier in traumatic injury and repair. CPD (2005) 11(11):1353–89. doi:10.2174/1381612053507837
41. Greitz, D. Unraveling the riddle of syringomyelia. Neurosurg Rev (2006) 29(4):251–64. doi:10.1007/s10143-006-0029-5
42. Reulen, HJ, Graham, R, Spatz, M, and Klatzo, I. Role of pressure gradients and bulk flow in dynamics of vasogenic brain edema. J Neurosurg (1977) 46(1):24–35. doi:10.3171/jns.1977.46.1.0024
43. Hilton, EL, and Henderson, LJ. Neurosurgical considerations in posttraumatic syringomyelia. AORN J (2003) 77(1):135–50. doi:10.1016/s0001-2092(06)61383-5
44. Berliner, J, Hemley, S, Najafi, E, Bilston, L, Stoodley, M, and Lam, M. Abnormalities in spinal cord ultrastructure in a rat model of post-traumatic syringomyelia. Fluids Barriers CNS (2020) 17(1):11. doi:10.1186/s12987-020-0171-4
45. Kigerl, KA, McGaughy, VM, and Popovich, PG. Comparative analysis of lesion development and intraspinal inflammation in four strains of mice following spinal contusion injury. J Comp Neurol (2006) 494(4):578–94. doi:10.1002/cne.20827
46. Donnelly, DJ, and Popovich, PG. Inflammation and its role in neuroprotection, axonal regeneration and functional recovery after spinal cord injury. Exp Neurol (2008) 209(2):378–88. doi:10.1016/j.expneurol.2007.06.009
47. Trivedi, A, Olivas, AD, and Noble-Haeusslein, LJ. Inflammation and spinal cord injury: infiltrating leukocytes as determinants of injury and repair processes. Clin Neurosci Res (2006) 6(5):283–92. doi:10.1016/j.cnr.2006.09.007
48. Hellenbrand, DJ, Quinn, CM, Piper, ZJ, Morehouse, CN, Fixel, JA, and Hanna, AS. Inflammation after spinal cord injury: a review of the critical timeline of signaling cues and cellular infiltration. J Neuroinflammation (2021) 18(1):284. doi:10.1186/s12974-021-02337-2
49. Beck, KD, Nguyen, HX, Galvan, MD, Salazar, DL, Woodruff, TM, and Anderson, AJ. Quantitative analysis of cellular inflammation after traumatic spinal cord injury: evidence for a multiphasic inflammatory response in the acute to chronic environment. Brain (2010) 133(2):433–47. doi:10.1093/brain/awp322
50. Sofroniew, MV. Molecular dissection of reactive astrogliosis and glial scar formation. Trends Neurosciences (2009) 32(12):638–47. doi:10.1016/j.tins.2009.08.002
51. David, S, and Kroner, A. Repertoire of microglial and macrophage responses after spinal cord injury. Nat Rev Neurosci (2011) 12(7):388–99. doi:10.1038/nrn3053
52. DiSabato, DJ, Marion, CM, Mifflin, KA, Alfredo, AN, Rodgers, KA, Kigerl, KA, et al. System failure: systemic inflammation following spinal cord injury. Eur J Immunol (2024) 54(1):2250274. doi:10.1002/eji.202250274
53. Gensel, JC, and Zhang, B. Macrophage activation and its role in repair and pathology after spinal cord injury. Brain Res (2015) 1619:1–11. doi:10.1016/j.brainres.2014.12.045
54. Mabon, PJ, Weaver, LC, and Dekaban, GA. Inhibition of monocyte/macrophage migration to a spinal cord injury site by an antibody to the integrin αD: a potential new anti-inflammatory treatment. Exp Neurol (2000) 166(1):52–64. doi:10.1006/exnr.2000.7488
55. Martinez, FO, Helming, L, and Gordon, S. Alternative activation of macrophages: an immunologic functional perspective. Annu Rev Immunol (2009) 27(1):451–83. doi:10.1146/annurev.immunol.021908.132532
56. Gensel, JC, Nakamura, S, Guan, Z, Van Rooijen, N, Ankeny, DP, and Popovich, PG. Macrophages promote axon regeneration with concurrent neurotoxicity. J Neurosci (2009) 29(12):3956–68. doi:10.1523/jneurosci.3992-08.2009
57. Amar, AP, and Levy, ML. Pathogenesis and pharmacological strategies for mitigating secondary damage in acute spinal cord injury. Neurosurgery (1999) 44(5):1027–39. doi:10.1097/00006123-199905000-00052
58. Sandler, AN, and Tator, CH. Effect of acute spinal cord compression injury on regional spinal cord blood flow in primates. J Neurosurg (1976) 45(6):660–76. doi:10.3171/jns.1976.45.6.0660
59. Yao, X, Feng, Sq, Hao, J, Li, B, Duan, Hq, Zhao, Cx, et al. Mechanisms underlying the promotion of functional recovery by deferoxamine after spinal cord injury in rats. Neural Regen Res (2017) 12(6):959. doi:10.4103/1673-5374.208591
60. Mortazavi, MM, Verma, K, Harmon, OA, Griessenauer, CJ, Adeeb, N, Theodore, N, et al. The microanatomy of spinal cord injury: a review. Clin Anat (2015) 28(1):27–36. doi:10.1002/ca.22432
61. Tran, AP, Warren, PM, and Silver, J. The biology of regeneration failure and success after spinal cord injury. Physiol Rev (2018) 98(2):881–917. doi:10.1152/physrev.00017.2017
62. Young, KM, Psachoulia, K, Tripathi, RB, Dunn, SJ, Cossell, L, Attwell, D, et al. Oligodendrocyte dynamics in the healthy adult CNS: evidence for myelin remodeling. Neuron (2013) 77(5):873–85. doi:10.1016/j.neuron.2013.01.006
63. Chong, SYC, Rosenberg, SS, Fancy, SPJ, Zhao, C, Shen, YAA, Hahn, AT, et al. Neurite outgrowth inhibitor Nogo-A establishes spatial segregation and extent of oligodendrocyte myelination. Proc Natl Acad Sci USA (2012) 109(4):1299–304. doi:10.1073/pnas.1113540109
64. McTigue, DM, Wei, P, and Stokes, BT. Proliferation of NG2-positive cells and altered oligodendrocyte numbers in the contused rat spinal cord. J Neurosci (2001) 21(10):3392–400. doi:10.1523/jneurosci.21-10-03392.2001
65. Blight, AR. Delayed demyelination and macrophage invasion: a candidate for secondary cell damage in spinal cord injury. Cent Nervous Syst Trauma (1985) 2(4):299–315. doi:10.1089/cns.1985.2.299
66. Powers, BE, Lasiene, J, Plemel, JR, Shupe, L, Perlmutter, SI, Tetzlaff, W, et al. Axonal thinning and extensive remyelination without chronic demyelination in spinal injured rats. J Neurosci (2012) 32(15):5120–5. doi:10.1523/jneurosci.0002-12.2012
67. Alizadeh, A, Dyck, SM, and Karimi-Abdolrezaee, S. Myelin damage and repair in pathologic CNS: challenges and prospects. Front Mol Neurosci (2015) 8:35. doi:10.3389/fnmol.2015.00035
68. Almad, A, Sahinkaya, FR, and McTigue, DM. Oligodendrocyte fate after spinal cord injury. Neurotherapeutics (2011) 8(2):262–73. doi:10.1007/s13311-011-0033-5
69. Kastin, A, and Pan, W. Targeting neurite growth inhibitors to induce CNS regeneration. CPD (2005) 11(10):1247–53. doi:10.2174/1381612053507440
70. Fan, B, Wei, Z, Yao, X, Shi, G, Cheng, X, Zhou, X, et al. Microenvironment imbalance of spinal cord injury. Cel Transpl (2018) 27(6):853–66. doi:10.1177/0963689718755778
71. Talifu, Z, Qin, C, Xin, Z, Chen, Y, Liu, J, Dangol, S, et al. The overexpression of insulin-like growth factor-1 and neurotrophin-3 promote functional recovery and alleviate spasticity after spinal cord injury. Front Neurosci (2022) 16:863793. doi:10.3389/fnins.2022.863793
72. Zhang, S, Meor Azlan, NF, Josiah, SS, Zhou, J, Zhou, X, Jie, L, et al. The role of SLC12A family of cation-chloride cotransporters and drug discovery methodologies. J Pharm Anal (2023) 13(12):1471–95. doi:10.1016/j.jpha.2023.09.002
73. Fakhri, S, Abbaszadeh, F, and Jorjani, M. On the therapeutic targets and pharmacological treatments for pain relief following spinal cord injury: a mechanistic review. Biomed and Pharmacother (2021) 139:111563. doi:10.1016/j.biopha.2021.111563
74. Boulenguez, P, Liabeuf, S, Bos, R, Bras, H, Jean-Xavier, C, Brocard, C, et al. Down-regulation of the potassium-chloride cotransporter KCC2 contributes to spasticity after spinal cord injury. Nat Med (2010) 16(3):302–7. doi:10.1038/nm.2107
75. Huang, YJ, Lee, KH, and Grau, JW. Complete spinal cord injury (SCI) transforms how brain derived neurotrophic factor (BDNF) affects nociceptive sensitization. Exp Neurol (2017) 288:38–50. doi:10.1016/j.expneurol.2016.11.001
76. Cramer, SW, Baggott, C, Cain, J, Tilghman, J, Allcock, B, Miranpuri, G, et al. The role of cation-dependent chloride transporters in neuropathic pain following spinal cord injury. Mol Pain (2008) 4:1744-8069–4-36. doi:10.1186/1744-8069-4-36
77. Rivera, C, Li, H, Thomas-Crusells, J, Lahtinen, H, Viitanen, T, Nanobashvili, A, et al. BDNF-induced TrkB activation down-regulates the K+-Cl-cotransporter KCC2 and impairs neuronal Cl-extrusion. J Cel Biol (2002) 159(5):747–52. doi:10.1083/jcb.200209011
78. Miletic, G, and Miletic, V. Loose ligation of the sciatic nerve is associated with TrkB receptor-dependent decreases in KCC2 protein levels in the ipsilateral spinal dorsal horn. Pain (2008) 137(3):532–9. doi:10.1016/j.pain.2007.10.016
79. Sivilotti, L, and Woolf, CJ. The contribution of GABAA and glycine receptors to central sensitization: disinhibition and touch-evoked allodynia in the spinal cord. J Neurophysiol (1994) 72(1):169–79. doi:10.1152/jn.1994.72.1.169
80. Huang, YJ, and Grau, JW. Ionic plasticity and pain: the loss of descending serotonergic fibers after spinal cord injury transforms how GABA affects pain. Exp Neurol (2018) 306:105–16. doi:10.1016/j.expneurol.2018.05.002
81. Bilchak, JN, Yeakle, K, Caron, G, Malloy, D, and Côté, MP. Enhancing KCC2 activity decreases hyperreflexia and spasticity after chronic spinal cord injury. Exp Neurol (2021) 338:113605. doi:10.1016/j.expneurol.2021.113605
82. Harvey, LA. Physiotherapy rehabilitation for people with spinal cord injuries. J Physiother (2016) 62(1):4–11. doi:10.1016/j.jphys.2015.11.004
83. Cote, MP, Gandhi, S, Zambrotta, M, and Houle, JD. Exercise modulates chloride homeostasis after spinal cord injury. J Neurosci (2014) 34(27):8976–87. doi:10.1523/jneurosci.0678-14.2014
84. Chen, B, Li, Y, Yu, B, Zhang, Z, Brommer, B, Williams, PR, et al. Reactivation of dormant relay pathways in injured spinal cord by KCC2 manipulations. Cell (2018) 174(3):521–35.e13. doi:10.1016/j.cell.2018.06.005
85. Gerteis, M, Edgman-Levitan, S, Walker, JD, Stoke, DM, Cleary, PD, and Delbanco, TL. What patients really want. Health Manage Q. (1993) 15(3):2–6.
86. Fehlings, MG, Tetreault, LA, Wilson, JR, Kwon, BK, Burns, AS, Martin, AR, et al. A clinical practice guideline for the management of acute spinal cord injury: introduction, rationale, and scope. Glob Spine J (2017) 7(3 Suppl. l):84S–94S. doi:10.1177/2192568217703387
87. Engkasan, JP, Ng, CJ, and Low, WY. Who decides? A qualitative study on the decisional roles of patients, their caregivers and doctors on the method of bladder drainage after spinal cord injury. Spinal Cord (2015) 53(2):130–4. doi:10.1038/sc.2014.199
88. Scheel-Sailer, A, Post, MW, Michel, F, Weidmann-Hügle, T, and Baumann Hölzle, R. Patients’ views on their decision making during inpatient rehabilitation after newly acquired spinal cord injury-A qualitative interview-based study. Health Expect (2017) 20(5):1133–42. doi:10.1111/hex.12559
89. Bowers, CA, Kundu, B, Rosenbluth, J, and Hawryluk, GWJ. Patients with spinal cord injuries favor administration of methylprednisolone. PLoS One (2016) 11(1):e0145991. doi:10.1371/journal.pone.0145991
90. Burkell, JA, Wolfe, DL, Potter, PJ, and Jutai, JW. Information needs and information sources of individuals living with spinal cord injury. Health Inf and Libraries J (2006) 23(4):257–65. doi:10.1111/j.1471-1842.2006.00686.x
91. Edwards, L, Krassioukov, A, and Fehlings, MG. Importance of access to research information among individuals with spinal cord injury: results of an evidenced-based questionnaire. Spinal Cord (2002) 40(10):529–35. doi:10.1038/sj.sc.3101364
92. Farrehi, C, Pazzi, C, Capron, M, Anderson, K, Richardson, B, and Stillman, M. How individuals with spinal cord injury in the United States access and assess information about experimental therapies and clinical trials: results of a clinical survey. Spinal Cord Ser Cases (2020) 6(1):103. doi:10.1038/s41394-020-00354-6
93. Yuen, J, Thiyagarajan, CA, and Belci, M. Patient experience survey in telemedicine for spinal cord injury patients. Spinal Cord (2015) 53(4):320–3. doi:10.1038/sc.2014.247
94. Bahsoun, S, Kuiper, JH, Hulme, CH, Twigg, AJA, El Masri, W, Glass, C, et al. Evaluating patient perspectives on participating in scientific research and clinical trials for the treatment of spinal cord injury. Sci Rep (2021) 11(1):4361. doi:10.1038/s41598-021-83211-2
95. Widerström-Noga, EG, Felipe-Cuervo, E, and Yezierski, RP. Chronic pain after spinal injury: interference with sleep and daily activities. Arch Phys Med Rehabil (2001) 82(11):1571–7. doi:10.1053/apmr.2001.26068
96. Jensen, MP, Kuehn, CM, Amtmann, D, and Cardenas, DD. Symptom burden in persons with spinal cord injury. Arch Phys Med Rehabil (2007) 88(5):638–45. doi:10.1016/j.apmr.2007.02.002
97. Burke, D, Fullen, BM, Stokes, D, and Lennon, O. Neuropathic pain prevalence following spinal cord injury: a systematic review and meta-analysis. Eur J Pain (2017) 21(1):29–44. doi:10.1002/ejp.905
98. Finnerup, NB, Johannesen, IL, Sindrup, SH, Bach, FW, and Jensen, TS. Pain and dysesthesia in patients with spinal cord injury: a postal survey. Spinal Cord (2001) 39(5):256–62. doi:10.1038/sj.sc.3101161
99. Sharif-Alhoseini, M, Khormali, M, Rezaei, M, Safdarian, M, Hajighadery, A, Khalatbari, MM, et al. Animal models of spinal cord injury: a systematic review. Spinal Cord (2017) 55(8):714–21. doi:10.1038/sc.2016.187
100. Badhiwala, JH, Wilson, JR, Witiw, CD, Harrop, JS, Vaccaro, AR, Aarabi, B, et al. The influence of timing of surgical decompression for acute spinal cord injury: a pooled analysis of individual patient data. Lancet Neurol (2021) 20(2):117–26. doi:10.1016/s1474-4422(20)30406-3
101. Fehlings, MG, Hachem, LD, Tetreault, LA, Skelly, AC, Dettori, JR, Brodt, ED, et al. Timing of decompressive surgery in patients with acute spinal cord injury: systematic review update. Glob Spine J (2024) 14(3_Suppl. l):38S–57S. doi:10.1177/21925682231197404
102. Hejrati, N, Moghaddamjou, A, Pedro, K, Alvi, MA, Harrop, JS, Guest, JD, et al. Current practice of acute spinal cord injury management: a global survey of members from the AO spine. Glob Spine J (2024) 14(2):546–60. doi:10.1177/21925682221116888
103. Guha, A, Tator, CH, Smith, CR, and Piper, I. Improvement in post-traumatic spinal cord blood flow with a combination of a calcium channel blocker and a vasopressor. J Trauma Inj Infect Crit Care (1989) 29(10):1440–7. doi:10.1097/00005373-198910000-00025
104. Fehlings, MG, Tator, CH, and Linden, RD. The effect of nimodipine and dextran on axonal function and blood flow following experimental spinal cord injury. J Neurosurg (1989) 71(3):403–16. doi:10.3171/jns.1989.71.3.0403
105. Ryken, TC, Hurlbert, RJ, Hadley, MN, Aarabi, B, Dhall, SS, Gelb, DE, et al. The acute cardiopulmonary management of patients with cervical spinal cord injuries. Neurosurgery (2013) 72(Suppl. 2):84–92. doi:10.1227/neu.0b013e318276ee16
106. Kwon, BK, Tetreault, LA, Martin, AR, Arnold, PM, Marco, RAW, Newcombe, VFJ, et al. A clinical practice guideline for the management of patients with acute spinal cord injury: recommendations on hemodynamic management. Glob Spine J (2024) 14(3_Suppl. l):187S–211S. doi:10.1177/21925682231202348
107. Squair, JW, Bélanger, LM, Tsang, A, Ritchie, L, Mac-Thiong, JM, Parent, S, et al. Spinal cord perfusion pressure predicts neurologic recovery in acute spinal cord injury. Neurology (2017) 89(16):1660–7. doi:10.1212/wnl.0000000000004519
108. Hall, ED, and Braughler, JM. Effects of intravenous methylprednisolone on spinal cord lipid peroxidation and Effects of intravenous methylprednisolone on spinal cord lipid peroxidation and (Na+ + K+)-ATPase activity: dose-response analysis during 1st hour after contusion injury in the cat. J Neurosurg (1982) 57(2):247–53. doi:10.3171/jns.1982.57.2.0247
109. Bracken, MB, Shepard, MJ, Collins, WF, Holford, TR, Young, W, Baskin, DS, et al. A randomized, controlled trial of methylprednisolone or naloxone in the treatment of acute spinal-cord injury. Results of the Second National Acute Spinal Cord Injury Study. N Engl J Med (1990) 322(20):1405–11. doi:10.1056/nejm199005173222001
110. Bracken, MB, Shepard, MJ, Holford, TR, Leo-Summers, L, Aldrich, EF, Fazl, M, et al. Administration of methylprednisolone for 24 or 48 hours or tirilazad mesylate for 48 hours in the treatment of acute spinal cord injury. Results of the third national acute spinal cord injury randomized controlled trial. National acute spinal cord injury study. JAMA (1997) 277(20):1597–604. doi:10.1001/jama.1997.03540440031029
111. Evaniew, N, Noonan, VK, Fallah, N, Kwon, BK, Rivers, CS, Ahn, H, et al. Methylprednisolone for the treatment of patients with acute spinal cord injuries: a propensity score-matched cohort study from a Canadian multi-center spinal cord injury Registry. J Neurotrauma (2015) 32(21):1674–83. doi:10.1089/neu.2015.3963
112. McNamee, LM, Walsh, MJ, and Ledley, FD. Timelines of translational science: from technology initiation to FDA approval. PLoS One (2017) 12(5):e0177371. doi:10.1371/journal.pone.0177371
113. Wichman, C, Smith, LM, and Yu, F. A framework for clinical and translational research in the era of rigor and reproducibility. J Clin Translational Sci (2020) 5(1):e31. doi:10.1017/cts.2020.523
114. Landi, M, Everitt, J, and Berridge, B. Bioethical, reproducibility, and translational challenges of animal models. ILAR J (2021) 62(1–2):60–5. doi:10.1093/ilar/ilaa027
115. Kjell, J, and Olson, L. Rat models of spinal cord injury: from pathology to potential therapies. Dis Models and Mech (2016) 9(10):1125–37. doi:10.1242/dmm.025833
116. Nagoshi, N, Khazaei, M, Ahlfors, JE, Ahuja, CS, Nori, S, Wang, J, et al. Human spinal oligodendrogenic neural progenitor cells promote functional recovery after spinal cord injury by axonal remyelination and tissue sparing. Stem Cell Translational Med (2018) 7(11):806–18. doi:10.1002/sctm.17-0269
117. Carpenter, RS, Kigerl, KA, Marbourg, JM, Gaudet, AD, Huey, D, Niewiesk, S, et al. Traumatic spinal cord injury in mice with human immune systems. Exp Neurol (2015) 271:432–44. doi:10.1016/j.expneurol.2015.07.011
118. Cheriyan, T, Ryan, DJ, Weinreb, JH, Cheriyan, J, Paul, JC, Lafage, V, et al. Spinal cord injury models: a review. Spinal Cord (2014) 52(8):588–95. doi:10.1038/sc.2014.91
119. Kwon, BK, Streijger, F, Hill, CE, Anderson, AJ, Bacon, M, Beattie, MS, et al. Large animal and primate models of spinal cord injury for the testing of novel therapies. Exp Neurol (2015) 269:154–68. doi:10.1016/j.expneurol.2015.04.008
120. Raineteau, O, Fouad, K, Noth, P, Thallmair, M, and Schwab, ME. Functional switch between motor tracts in the presence of the mAb IN-1 in the adult rat. Proc Natl Acad Sci U S A (2001) 98(12):6929–34. doi:10.1073/pnas.111165498
121. Wild, BM, Mohan, R, and Morris, R. Rat motor neurons caudal to a rubrospinal tract (RST) transection remain viable. Neuroscience (2017) 364:157–63. doi:10.1016/j.neuroscience.2017.09.013
122. Lee, JHT, Jones, CF, Okon, EB, Anderson, L, Tigchelaar, S, Kooner, P, et al. A novel porcine model of traumatic thoracic spinal cord injury. J Neurotrauma (2013) 30(3):142–59. doi:10.1089/neu.2012.2386
123. Weber-Levine, C, Hersh, AM, Jiang, K, Routkevitch, D, Tsehay, Y, Perdomo-Pantoja, A, et al. Porcine model of spinal cord injury: a systematic review. Neurotrauma Rep (2022) 3(1):352–68. doi:10.1089/neur.2022.0038
124. Lim, JH, Jung, CS, Byeon, YE, Kim, WH, Yoon, JH, Kang, KS, et al. Establishment of a canine spinal cord injury model induced by epidural balloon compression. J Vet Sci (2007) 8(1):311–94. doi:10.4142/jvs.2007.8.3.311
125. Goto, T, and Hoshino, Y. Electrophysiological, histological, and behavioral studies in a cat with acute compression of the spinal cord. J Orthopaedic Sci (2001) 6(1):59–67. doi:10.1007/s007760170026
126. Ma, Z, Zhang, YP, Liu, W, Yan, G, Li, Y, Shields, LBE, et al. A controlled spinal cord contusion for the rhesus macaque monkey. Exp Neurol (2016) 279:261–73. doi:10.1016/j.expneurol.2016.02.008
127. Gruner, JA. A monitored contusion model of spinal cord injury in the rat. J Neurotrauma (1992) 9(2):123–8. discussion 126-128. doi:10.1089/neu.1992.9.123
128. Scheff, SW, Rabchevsky, AG, Fugaccia, I, Main, JA, and Lumpp, JE. Experimental modeling of spinal cord injury: characterization of a force-defined injury device. J Neurotrauma (2003) 20(2):179–93. doi:10.1089/08977150360547099
129. Rivlin, AS, and Tator, CH. Effect of duration of acute spinal cord compression in a new acute cord injury model in the rat. Surg Neurol (1978) 10(1):38–43.
130. Blight, AR. Morphometric analysis of a model of spinal cord injury in Guinea pigs, with behavioral evidence of delayed secondary pathology. J Neurol Sci (1991) 103(2):156–71. doi:10.1016/0022-510x(91)90159-5
131. Tarlov, IM, Klinger, H, and Vitale, S. Spinal cord compression studies. I. Experimental techniques to produce acute and gradual compression. AMA Arch Neurol Psychiatry (1953) 70(6):813–9. doi:10.1001/archneurpsyc.1953.02320360128010
132. Reinhardt, DR, Stehlik, KE, Satkunendrarajah, K, and Kroner, A. Bilateral cervical contusion spinal cord injury: a mouse model to evaluate sensorimotor function. Exp Neurol (2020) 331:113381. doi:10.1016/j.expneurol.2020.113381
133. Wilcox, JT, Satkunendrarajah, K, Nasirzadeh, Y, Laliberte, AM, Lip, A, Cadotte, DW, et al. Generating level-dependent models of cervical and thoracic spinal cord injury: exploring the interplay of neuroanatomy, physiology, and function. Neurobiol Dis (2017) 105:194–212. doi:10.1016/j.nbd.2017.05.009
134. Hong, J, Chang, A, Zavvarian, MM, Wang, J, Liu, Y, and Fehlings, MG. Level-specific differences in systemic expression of pro- and anti-inflammatory cytokines and chemokines after spinal cord injury. Int J Mol Sci (2018) 19(8):2167. doi:10.3390/ijms19082167
135. Seyhan, AA. Lost in translation: the valley of death across preclinical and clinical divide – identification of problems and overcoming obstacles. Translational Med Commun (2019) 4(1):18. doi:10.1186/s41231-019-0050-7
136. Barnabé-Heider, F, Göritz, C, Sabelström, H, Takebayashi, H, Pfrieger, FW, Meletis, K, et al. Origin of new glial cells in intact and injured adult spinal cord. Cell Stem Cell (2010) 7(4):470–82. doi:10.1016/j.stem.2010.07.014
137. Stenudd, M, Sabelström, H, and Frisén, J. Role of endogenous neural stem cells in spinal cord injury and repair. JAMA Neurol (2015) 72(2):235–7. doi:10.1001/jamaneurol.2014.2927
138. Sabelström, H, Stenudd, M, Réu, P, Dias, DO, Elfineh, M, Zdunek, S, et al. Resident neural stem cells restrict tissue damage and neuronal loss after spinal cord injury in mice. Science (2013) 342(6158):637–40. doi:10.1126/science.1242576
139. Zhang, C, Dong, P, Bai, Y, and Quan, D. Nanofibrous polyester-polypeptide block copolymer scaffolds with high porosity and controlled degradation promote cell adhesion, proliferation and differentiation. Eur Polym J (2020) 130:109647. doi:10.1016/j.eurpolymj.2020.109647
140. Ohori, Y, Yamamoto, Sichi, Nagao, M, Sugimori, M, Yamamoto, N, Nakamura, K, et al. Growth factor treatment and genetic manipulation stimulate neurogenesis and oligodendrogenesis by endogenous neural progenitors in the injured adult spinal cord. J Neurosci (2006) 26(46):11948–60. doi:10.1523/jneurosci.3127-06.2006
141. Khazaei, M, Ahuja, CS, Rodgers, CE, Chan, P, and Fehlings, MG. Generation of definitive neural progenitor cells from human pluripotent stem cells for transplantation into spinal cord injury. In: MM Daadi, editor. Neural stem cells: methods and protocols. New York, NY: Springer (2019). p. 25–41. doi:10.1007/978-1-4939-9007-8_3
142. Takahashi, K, and Yamanaka, S. Induction of pluripotent stem cells from mouse embryonic and adult fibroblast cultures by defined factors. Cell (2006) 126(4):663–76. doi:10.1016/j.cell.2006.07.024
143. Takahashi, K, Tanabe, K, Ohnuki, M, Narita, M, Ichisaka, T, Tomoda, K, et al. Induction of pluripotent stem cells from adult human fibroblasts by defined factors. Cell (2007) 131(5):861–72. doi:10.1016/j.cell.2007.11.019
144. Nishimori, M, Yakushiji, H, Mori, M, Miyamoto, T, Yaguchi, T, Ohno, S, et al. Tumorigenesis in cells derived from induced pluripotent stem cells. Hum Cel (2014) 27(1):29–35. doi:10.1007/s13577-013-0078-3
145. Dang, CV, Le, A, and Gao, P. MYC-induced cancer cell energy metabolism and therapeutic opportunities. Clin Cancer Res (2009) 15(21):6479–83. doi:10.1158/1078-0432.ccr-09-0889
146. Zhao, T, Zhang, ZN, Rong, Z, and Xu, Y. Immunogenicity of induced pluripotent stem cells. Nature (2011) 474(7350):212–5. doi:10.1038/nature10135
147. Okita, K, Ichisaka, T, and Yamanaka, S. Generation of germline-competent induced pluripotent stem cells. Nature (2007) 448(7151):313–7. doi:10.1038/nature05934
148. Nakagawa, M, Koyanagi, M, Tanabe, K, Takahashi, K, Ichisaka, T, Aoi, T, et al. Generation of induced pluripotent stem cells without Myc from mouse and human fibroblasts. Nat Biotechnol (2008) 26(1):101–6. doi:10.1038/nbt1374
149. Chhabra, A. Derivation of human induced pluripotent stem cell (iPSC) lines and mechanism of pluripotency: historical perspective and recent advances. Stem Cel Rev Rep (2017) 13(6):757–73. doi:10.1007/s12015-017-9766-9
150. Ban, H, Nishishita, N, Fusaki, N, Tabata, T, Saeki, K, Shikamura, M, et al. Efficient generation of transgene-free human induced pluripotent stem cells (iPSCs) by temperature-sensitive Sendai virus vectors. Proc Natl Acad Sci (2011) 108(34):14234–9. doi:10.1073/pnas.1103509108
151. Okita, K, Nakagawa, M, Hyenjong, H, Ichisaka, T, and Yamanaka, S. Generation of mouse induced pluripotent stem cells without viral vectors. Science. (2008) 322(5903):949–53. doi:10.1126/science.1164270
152. Zhou, W, and Freed, CR. Adenoviral gene delivery can reprogram human fibroblasts to induced pluripotent stem cells. Stem Cells (2009) 27(11):2667–74. doi:10.1002/stem.201
153. Warren, L, Manos, PD, Ahfeldt, T, Loh, YH, Li, H, Lau, F, et al. Highly efficient reprogramming to pluripotency and directed differentiation of human cells with synthetic modified mRNA. Cell Stem Cell (2010) 7(5):618–30. doi:10.1016/j.stem.2010.08.012
154. Warren, L, and Lin, C. mRNA-based genetic reprogramming. Mol Ther (2019) 27(4):729–34. doi:10.1016/j.ymthe.2018.12.009
155. Ahlfors, JE, Azimi, A, El-Ayoubi, R, Velumian, A, Vonderwalde, I, Boscher, C, et al. Examining the fundamental biology of a novel population of directly reprogrammed human neural precursor cells. Stem Cel Res Ther (2019) 10(1):166. doi:10.1186/s13287-019-1255-4
156. Casha, S, Zygun, D, McGowan, MD, Bains, I, Yong, VW, and John Hurlbert, R. Results of a phase II placebo-controlled randomized trial of minocycline in acute spinal cord injury. Brain (2012) 135(Pt 4):1224–36. doi:10.1093/brain/aws072
157. Fehlings, MG, Moghaddamjou, A, Harrop, JS, Stanford, R, Ball, J, Aarabi, B, et al. Safety and efficacy of riluzole in acute spinal cord injury study (RISCIS): a multi-center, randomized, placebo-controlled, double-blinded trial. J Neurotrauma (2023) 40(17–18):1878–88. doi:10.1089/neu.2023.0163
158. Koda, M, Hanaoka, H, Fujii, Y, Hanawa, M, Kawasaki, Y, Ozawa, Y, et al. Randomized trial of granulocyte colony-stimulating factor for spinal cord injury. Brain (2021) 144(3):789–99. doi:10.1093/brain/awaa466
159. Nagoshi, N, Tsuji, O, Kitamura, K, Suda, K, Maeda, T, Yato, Y, et al. Phase I/II study of intrathecal administration of recombinant human hepatocyte growth factor in patients with acute spinal cord injury: a double-blind, randomized clinical trial of safety and efficacy. J Neurotrauma (2020) 37(15):1752–8. doi:10.1089/neu.2019.6854
160. Wells, JEA, Hurlbert, RJ, Fehlings, MG, and Yong, VW. Neuroprotection by minocycline facilitates significant recovery from spinal cord injury in mice. Brain (2003) 126(Pt 7):1628–37. doi:10.1093/brain/awg178
161. Festoff, BW, Ameenuddin, S, Arnold, PM, Wong, A, Santacruz, KS, and Citron, BA. Minocycline neuroprotects, reduces microgliosis, and inhibits caspase protease expression early after spinal cord injury. J Neurochem (2006) 97(5):1314–26. doi:10.1111/j.1471-4159.2006.03799.x
162. Nishio, Y, Koda, M, Kamada, T, Someya, Y, Kadota, R, Mannoji, C, et al. Granulocyte colony-stimulating factor attenuates neuronal death and promotes functional recovery after spinal cord injury in mice. J Neuropathol Exp Neurol (2007) 66(8):724–31. doi:10.1097/nen.0b013e3181257176
163. Kawabe, J, Koda, M, Hashimoto, M, Fujiyoshi, T, Furuya, T, Endo, T, et al. Neuroprotective effects of granulocyte colony-stimulating factor and relationship to promotion of angiogenesis after spinal cord injury in rats: laboratory investigation. J Neurosurg Spine (2011) 15(4):414–21. doi:10.3171/2011.5.spine10421
164. Kadota, R, Koda, M, Kawabe, J, Hashimoto, M, Nishio, Y, Mannoji, C, et al. Granulocyte colony-stimulating factor (G-CSF) protects oligpdendrocyte and promotes hindlimb functional recovery after spinal cord injury in rats. PLoS One (2012) 7(11):e50391. doi:10.1371/journal.pone.0050391
165. Takahashi, H, Yamazaki, M, Okawa, A, Sakuma, T, Kato, K, Hashimoto, M, et al. Neuroprotective therapy using granulocyte colony-stimulating factor for acute spinal cord injury: a phase I/IIa clinical trial. Eur Spine J (2012) 21(12):2580–7. doi:10.1007/s00586-012-2213-3
166. Inada, T, Takahashi, H, Yamazaki, M, Okawa, A, Sakuma, T, Kato, K, et al. Multicenter prospective nonrandomized controlled clinical trial to prove neurotherapeutic effects of granulocyte colony-stimulating factor for acute spinal cord injury: analyses of follow-up cases after at least 1 year. Spine (Phila Pa 1976) (2014) 39(3):213–9. doi:10.1097/brs.0000000000000121
167. Kitamura, K, Iwanami, A, Nakamura, M, Yamane, J, Watanabe, K, Suzuki, Y, et al. Hepatocyte growth factor promotes endogenous repair and functional recovery after spinal cord injury. J Neurosci Res (2007) 85(11):2332–42. doi:10.1002/jnr.21372
168. Fessler, RG, Ehsanian, R, Liu, CY, Steinberg, GK, Jones, L, Lebkowski, JS, et al. A phase 1/2a dose-escalation study of oligodendrocyte progenitor cells in individuals with subacute cervical spinal cord injury. J Neurosurg Spine (2022) 37(6):812–20. doi:10.3171/2022.5.spine22167
169. Honmou, O, Yamashita, T, Morita, T, Oshigiri, T, Hirota, R, Iyama, S, et al. Intravenous infusion of auto serum-expanded autologous mesenchymal stem cells in spinal cord injury patients: 13 case series. Clin Neurol Neurosurg (2021) 203:106565. doi:10.1016/j.clineuro.2021.106565
170. Bydon, M, Qu, W, Moinuddin, FM, Hunt, CL, Garlanger, KL, Reeves, RK, et al. Intrathecal delivery of adipose-derived mesenchymal stem cells in traumatic spinal cord injury: phase I trial. Nat Commun (2024) 15(1):2201. doi:10.1038/s41467-024-46259-y
171. Fehlings, MG, Chen, Y, Aarabi, B, Ahmad, F, Anderson, KD, Dumont, T, et al. A randomized controlled trial of local delivery of a Rho inhibitor (VX-210) in patients with acute traumatic cervical spinal cord injury. J Neurotrauma (2021) 38(15):2065–72. doi:10.1089/neu.2020.7096
172. Ghobrial, GM, Anderson, KD, Dididze, M, Martinez-Barrizonte, J, Sunn, GH, Gant, KL, et al. Human neural stem cell transplantation in chronic cervical spinal cord injury: functional outcomes at 12 Months in a phase II clinical trial. Neurosurgery (2017) 64(CN_Suppl. l_1):87–91. doi:10.1093/neuros/nyx242
173. Levi, AD, Okonkwo, DO, Park, P, Jenkins, AL, Kurpad, SN, Parr, AM, et al. Emerging safety of intramedullary transplantation of human neural stem cells in chronic cervical and thoracic spinal cord injury. Neurosurgery (2018) 82(4):562–75. doi:10.1093/neuros/nyx250
174. Levi, AD, Anderson, KD, Okonkwo, DO, Park, P, Bryce, TN, Kurpad, SN, et al. Clinical outcomes from a multi-center study of human neural stem cell transplantation in chronic cervical spinal cord injury. J Neurotrauma (2019) 36(6):891–902. doi:10.1089/neu.2018.5843
175. Martin, J, Cleary, D, Mendoza, M, Cabrera, B, Jamieson, CHM, Marsala, M, et al. Long-term clinical and safety outcomes from a single-site phase I study of neural stem cell transplantation for chronic thoracic spinal cord injury. Cell Stem Cell (2023). Available from: https://papers.ssrn.com/abstract=4501711 (Accessed May 4, 2024). doi:10.2139/ssrn.4501711
176. Sugai, K, Sumida, M, Shofuda, T, Yamaguchi, R, Tamura, T, Kohzuki, T, et al. First-in-human clinical trial of transplantation of iPSC-derived NS/PCs in subacute complete spinal cord injury: study protocol. Regenerative Ther (2021) 18:321–33. doi:10.1016/j.reth.2021.08.005
177. Keirstead, HS, Nistor, G, Bernal, G, Totoiu, M, Cloutier, F, Sharp, K, et al. Human embryonic stem cell-derived oligodendrocyte progenitor cell transplants remyelinate and restore locomotion after spinal cord injury. J Neurosci (2005) 25(19):4694–705. doi:10.1523/jneurosci.0311-05.2005
178. Zhang, YW, Denham, J, and Thies, RS. Oligodendrocyte progenitor cells derived from human embryonic stem cells express neurotrophic factors. Stem Cell Develop (2006) 15(6):943–52. doi:10.1089/scd.2006.15.943
179. Sharp, J, Frame, J, Siegenthaler, M, Nistor, G, and Keirstead, HS. Human embryonic stem cell-derived oligodendrocyte progenitor cell transplants improve recovery after cervical spinal cord injury. Stem Cells (2010) 28(1):152–63. doi:10.1002/stem.245
180. Liu, B, Wang, Yy, Wang, Hf, Liu, Xk, Li, R, Zhang, P, et al. Effect of glial cells on remyelination after spinal cord injury. Neural Regen Res (2017) 12(10):1724–32. doi:10.4103/1673-5374.217354
181. McKenna, SL, Ehsanian, R, Liu, CY, Steinberg, GK, Jones, L, Lebkowski, JS, et al. Ten-year safety of pluripotent stem cell transplantation in acute thoracic spinal cord injury. J Neurosurg Spine (2022) 37:321–30. doi:10.3171/2021.12.spine21622
182. Felts, PA, and Smith, KJ. Conduction properties of central nerve fibers remyelinated by Schwann cells. Brain Res (1992) 574(1–2):178–92. doi:10.1016/0006-8993(92)90815-q
183. Barbour, HR, Plant, CD, Harvey, AR, and Plant, GW. Tissue sparing, behavioral recovery, supraspinal axonal sparing/regeneration following sub-acute glial transplantation in a model of spinal cord contusion. BMC Neurosci (2013) 14:106. doi:10.1186/1471-2202-14-106
184. Pearse, DD, Bastidas, J, Izabel, SS, and Ghosh, M. Schwann cell transplantation subdues the pro-inflammatory innate immune cell response after spinal cord injury. Int J Mol Sci (2018) 19(9):2550. doi:10.3390/ijms19092550
185. Anderson, KD, Guest, JD, Dietrich, WD, Bartlett Bunge, M, Curiel, R, Dididze, M, et al. Safety of autologous human schwann cell transplantation in subacute thoracic spinal cord injury. J Neurotrauma (2017) 34(21):2950–63. doi:10.1089/neu.2016.4895
186. Maher, JL, Anderson, KD, Gant, KL, and Cowan, RE. Development and deployment of an at-home strength and conditioning program to support a phase I trial in persons with chronic spinal cord injury. Spinal Cord (2021) 59(1):44–54. doi:10.1038/s41393-020-0486-7
187. Cofano, F, Boido, M, Monticelli, M, Zenga, F, Ducati, A, Vercelli, A, et al. Mesenchymal stem cells for spinal cord injury: current options, limitations, and future of cell therapy. Int J Mol Sci (2019) 20(11):2698. doi:10.3390/ijms20112698
188. Filippi, M, Boido, M, Pasquino, C, Garello, F, Boffa, C, and Terreno, E. Successful in vivo MRI tracking of MSCs labeled with gadoteridol in a spinal cord injury experimental model. Exp Neurol (2016) 282:66–77. doi:10.1016/j.expneurol.2016.05.023
189. Pal, R, Gopinath, C, Rao, NM, Banerjee, P, Krishnamoorthy, V, Venkataramana, NK, et al. Functional recovery after transplantation of bone marrow-derived human mesenchymal stromal cells in a rat model of spinal cord injury. Cytotherapy (2010) 12(6):792–806. doi:10.3109/14653249.2010.487899
190. Nishio, Y, Koda, M, Kamada, T, Someya, Y, Yoshinaga, K, Okada, S, et al. The use of hemopoietic stem cells derived from human umbilical cord blood to promote restoration of spinal cord tissue and recovery of hindlimb function in adult rats. J Neurosurg Spine (2006) 5(5):424–33. doi:10.3171/spi.2006.5.5.424
191. Kim, Y, Jo, SH, Kim, WH, and Kweon, OK. Antioxidant and anti-inflammatory effects of intravenously injected adipose derived mesenchymal stem cells in dogs with acute spinal cord injury. Stem Cel Res Ther (2015) 6:229. doi:10.1186/s13287-015-0236-5
192. Ryu, HH, Kang, BJ, Park, SS, Kim, Y, Sung, GJ, Woo, HM, et al. Comparison of mesenchymal stem cells derived from fat, bone marrow, Wharton’s jelly, and umbilical cord blood for treating spinal cord injuries in dogs. J Vet Med Sci (2012) 74(12):1617–30. doi:10.1292/jvms.12-0065
193. Takamiya, S, Kawabori, M, Yamazaki, K, Yamaguchi, S, Tanimori, A, Yamamoto, K, et al. Intravenous transplantation of amnion-derived mesenchymal stem cells promotes functional recovery and alleviates intestinal dysfunction after spinal cord injury. PLOS ONE (2022) 17(7):e0270606. doi:10.1371/journal.pone.0270606
194. Smirnov, VA, Radaev, SM, Morozova, YV, Ryabov, SI, Yadgarov, MY, Bazanovich, SA, et al. Systemic administration of allogeneic cord blood mononuclear cells in adults with severe acute contusion spinal cord injury: phase 1/2a pilot clinical study-safety and primary efficacy evaluation. World Neurosurg (2022) 161:e319–38. doi:10.1016/j.wneu.2022.02.004
195. Dezawa, M. The Muse cell discovery, thanks to wine and science. Adv Exp Med Biol (2018) 1103:1–11. doi:10.1007/978-4-431-56847-6_1
196. Kajitani, T, Endo, T, Iwabuchi, N, Inoue, T, Takahashi, Y, Abe, T, et al. Association of intravenous administration of human Muse cells with deficit amelioration in a rat model of spinal cord injury. J Neurosurg Spine (2021) 34(4):648–55. doi:10.3171/2020.7.spine20293
197. Dergham, P, Ellezam, B, Essagian, C, Avedissian, H, Lubell, WD, and McKerracher, L. Rho signaling pathway targeted to promote spinal cord repair. J Neurosci (2002) 22(15):6570–7. doi:10.1523/jneurosci.22-15-06570.2002
198. Lord-Fontaine, S, Yang, F, Diep, Q, Dergham, P, Munzer, S, Tremblay, P, et al. Local inhibition of Rho signaling by cell-permeable recombinant protein BA-210 prevents secondary damage and promotes functional recovery following acute spinal cord injury. J Neurotrauma (2008) 25(11):1309–22. doi:10.1089/neu.2008.0613
199. Fehlings, MG, Theodore, N, Harrop, J, Maurais, G, Kuntz, C, Shaffrey, CI, et al. A phase I/IIa clinical trial of a recombinant Rho protein antagonist in acute spinal cord injury. J Neurotrauma (2011) 28(5):787–96. doi:10.1089/neu.2011.1765
200. Liu, J, Gao, HY, and Wang, XF. The role of the Rho/ROCK signaling pathway in inhibiting axonal regeneration in the central nervous system. Neural Regen Res (2015) 10(11):1892–6. doi:10.4103/1673-5374.170325
201. Freund, P, Schmidlin, E, Wannier, T, Bloch, J, Mir, A, Schwab, ME, et al. Nogo-A-specific antibody treatment enhances sprouting and functional recovery after cervical lesion in adult primates. Nat Med (2006) 12(7):790–2. doi:10.1038/nm1436
202. Kucher, K, Johns, D, Maier, D, Abel, R, Badke, A, Baron, H, et al. First-in-Man intrathecal application of neurite growth-promoting anti-nogo-A antibodies in acute spinal cord injury. Neurorehabil Neural Repair (2018) 32(6–7):578–89. doi:10.1177/1545968318776371
203. Maynard, G, Kannan, R, Liu, J, Wang, W, Lam, TKT, Wang, X, et al. Soluble Nogo-Receptor-Fc decoy (AXER-204) in patients with chronic cervical spinal cord injury in the USA: a first-in-human and randomised clinical trial. Lancet Neurol (2023) 22(8):672–84. doi:10.1016/s1474-4422(23)00215-6
204. Briyal, S, Ranjan, AK, Hornick, MG, Puppala, AK, Luu, T, and Gulati, A. Anti-apoptotic activity of ETB receptor agonist, IRL-1620, protects neural cells in rats with cerebral ischemia. Sci Rep (2019) 9(1):10439. doi:10.1038/s41598-019-46203-x
205. Ranjan, AK, Briyal, S, and Gulati, A. Sovateltide (IRL-1620) activates neuronal differentiation and prevents mitochondrial dysfunction in adult mammalian brains following stroke. Sci Rep (2020) 10(1):12737. doi:10.1038/s41598-020-69673-w
206. Gulati, A, Agrawal, N, Vibha, D, Misra, UK, Paul, B, Jain, D, et al. Safety and efficacy of Sovateltide (IRL-1620) in a multicenter randomized controlled clinical trial in patients with acute cerebral ischemic stroke. CNS Drugs (2021) 35(1):85–104. doi:10.1007/s40263-020-00783-9
207. Hata, K, Fujitani, M, Yasuda, Y, Doya, H, Saito, T, Yamagishi, S, et al. RGMa inhibition promotes axonal growth and recovery after spinal cord injury. J Cel Biol (2006) 173(1):47–58. doi:10.1083/jcb.200508143
208. Nakagawa, H, Ninomiya, T, Yamashita, T, and Takada, M. Treatment with the neutralizing antibody against repulsive guidance molecule-a promotes recovery from impaired manual dexterity in a primate model of spinal cord injury. Cereb Cortex (2019) 29(2):561–72. doi:10.1093/cercor/bhx338
209. Khan, NR, Smalley, Z, Nesvick, CL, Lee, SL, and Michael, LM. The use of lumbar drains in preventing spinal cord injury following thoracoabdominal aortic aneurysm repair: an updated systematic review and meta-analysis. J Neurosurg Spine (2016) 25(3):383–93. doi:10.3171/2016.1.spine151199
210. Kwon, BK, Curt, A, Belanger, LM, Bernardo, A, Chan, D, Markez, JA, et al. Intrathecal pressure monitoring and cerebrospinal fluid drainage in acute spinal cord injury: a prospective randomized trial. J Neurosurg Spine (2009) 10(3):181–93. doi:10.3171/2008.10.spine08217
211. Panchal, AR, Bartos, JA, Cabañas, JG, Donnino, MW, Drennan, IR, Hirsch, KG, et al. Part 3: adult basic and advanced life support: 2020 American Heart association guidelines for cardiopulmonary resuscitation and emergency cardiovascular care. Circulation. 2020;142(Suppl. l_2):S366–S468. doi:10.1161/CIR.0000000000000916
212. Batchelor, PE, Skeers, P, Antonic, A, Wills, TE, Howells, DW, Macleod, MR, et al. Systematic review and meta-analysis of therapeutic hypothermia in animal models of spinal cord injury. PLoS One (2013) 8(8):e71317. doi:10.1371/journal.pone.0071317
213. Ransom, SC, Brown, NJ, Pennington, ZA, Lakomkin, N, Mikula, AL, Bydon, M, et al. Hypothermia therapy for traumatic spinal cord injury: an updated review. J Clin Med (2022) 11(6):1585. doi:10.3390/jcm11061585
214. Vedantam, A, Jimsheleishvili, G, Harrop, JS, Alberga, LR, Ahmad, FU, Murphy, RK, et al. A prospective multi-center study comparing the complication profile of modest systemic hypothermia versus normothermia for acute cervical spinal cord injury. Spinal Cord (2022) 60(6):510–5. doi:10.1038/s41393-021-00747-w
215. Nagoshi, N, Nakashima, H, and Fehlings, MG. Riluzole as a neuroprotective drug for spinal cord injury: from bench to bedside. Molecules (2015) 20(5):7775–89. doi:10.3390/molecules20057775
216. Agrawal, SK, and Fehlings, MG. Mechanisms of secondary injury to spinal cord axons in vitro: role of Na+, Na(+)-K(+)-ATPase, the Na(+)-H+ exchanger, and the Na(+)-Ca2+ exchanger. J Neurosci (1996) 16(2):545–52. doi:10.1523/jneurosci.16-02-00545.1996
217. Satkunendrarajah, K, Nassiri, F, Karadimas, SK, Lip, A, Yao, G, and Fehlings, MG. Riluzole promotes motor and respiratory recovery associated with enhanced neuronal survival and function following high cervical spinal hemisection. Exp Neurol (2016) 276:59–71. doi:10.1016/j.expneurol.2015.09.011
218. Wu, Y, Satkunendrarajah, K, Teng, Y, Chow, DSL, Buttigieg, J, and Fehlings, MG. Delayed post-injury administration of riluzole is neuroprotective in a preclinical rodent model of cervical spinal cord injury. J Neurotrauma (2013) 30(6):441–52. doi:10.1089/neu.2012.2622
219. Grossman, RG, Fehlings, MG, Frankowski, RF, Burau, KD, Chow, DSL, Tator, C, et al. A prospective, multicenter, phase I matched-comparison group trial of safety, pharmacokinetics, and preliminary efficacy of riluzole in patients with traumatic spinal cord injury. J Neurotrauma (2014) 31(3):239–55. doi:10.1089/neu.2013.2969
220. Fehlings, MG, Badhiwala, JH, Ahn, H, Farhadi, HF, Shaffrey, CI, Nassr, A, et al. Safety and efficacy of riluzole in patients undergoing decompressive surgery for degenerative cervical myelopathy (CSM-Protect): a multicentre, double-blind, placebo-controlled, randomised, phase 3 trial. Lancet Neurol (2021) 20(2):98–106. doi:10.1016/s1474-4422(20)30407-5
221. Tazoe, T, and Perez, MA. Effects of repetitive transcranial magnetic stimulation on recovery of function after spinal cord injury. Arch Phys Med Rehabil (2015) 96(4):S145–55. doi:10.1016/j.apmr.2014.07.418
222. Gersner, R, Kravetz, E, Feil, J, Pell, G, and Zangen, A. Long-term effects of repetitive transcranial magnetic stimulation on markers for neuroplasticity: differential outcomes in anesthetized and awake animals. J Neurosci (2011) 31(20):7521–6. doi:10.1523/jneurosci.6751-10.2011
223. Fritsch, B, Reis, J, Martinowich, K, Schambra, HM, Ji, Y, Cohen, LG, et al. Direct current stimulation promotes BDNF-dependent synaptic plasticity: potential implications for motor learning. Neuron (2010) 66(2):198–204. doi:10.1016/j.neuron.2010.03.035
224. Kuppuswamy, A, Balasubramaniam, AV, Maksimovic, R, Mathias, CJ, Gall, A, Craggs, MD, et al. Action of 5 Hz repetitive transcranial magnetic stimulation on sensory, motor and autonomic function in human spinal cord injury. Clin Neurophysiol (2011) 122(12):2452–61. doi:10.1016/j.clinph.2011.04.022
225. André-Obadia, N, Peyron, R, Mertens, P, Mauguière, F, Laurent, B, and Garcia-Larrea, L. Transcranial magnetic stimulation for pain control. Double-blind study of different frequencies against placebo, and correlation with motor cortex stimulation efficacy. Clin Neurophysiol (2006) 117(7):1536–44. doi:10.1016/j.clinph.2006.03.025
226. Defrin, R, Grunhaus, L, Zamir, D, and Zeilig, G. The effect of a series of repetitive transcranial magnetic stimulations of the motor cortex on central pain after spinal cord injury. Arch Phys Med Rehabil (2007) 88(12):1574–80. doi:10.1016/j.apmr.2007.07.025
227. Khedr, EM. Longlasting antalgic effects of daily sessions of repetitive transcranial magnetic stimulation in central and peripheral neuropathic pain. J Neurol Neurosurg and Psychiatry (2005) 76(6):833–8. doi:10.1136/jnnp.2004.055806
228. Lefaucheur, JP. Neurogenic pain relief by repetitive transcranial magnetic cortical stimulation depends on the origin and the site of pain. J Neurol Neurosurg and Psychiatry (2004) 75(4):612–6. doi:10.1136/jnnp.2003.022236
229. Wincek, A, Huber, J, Leszczyńska, K, Fortuna, W, Okurowski, S, Chmielak, K, et al. The long-term effect of treatment using the transcranial magnetic stimulation rTMS in patients after incomplete cervical or thoracic spinal cord injury. JCM (2021) 10(13):2975. doi:10.3390/jcm10132975
230. Hofstoetter, US, Freundl, B, Danner, SM, Krenn, MJ, Mayr, W, Binder, H, et al. Transcutaneous spinal cord stimulation induces temporary attenuation of spasticity in individuals with spinal cord injury. J Neurotrauma (2020) 37(3):481–93. doi:10.1089/neu.2019.6588
231. Moritz, C, Field-Fote, EC, Tefertiller, C, van Nes, I, Trumbower, R, Kalsi-Ryan, S, et al. Non-invasive spinal cord electrical stimulation for arm and hand function in chronic tetraplegia: a safety and efficacy trial. Nat Med (2024) 30(5):1276–83. doi:10.1038/s41591-024-02940-9
232. Durán, FS, Lugo, L, Ramírez, L, and Lic, EE. Effects of an exercise program on the rehabilitation of patients with spinal cord injury. Arch Phys Med Rehabil (2001) 82(10):1349–54. doi:10.1053/apmr.2001.26066
233. Angeli, C, Rejc, E, Boakye, M, Herrity, A, Mesbah, S, Hubscher, C, et al. Targeted selection of stimulation parameters for restoration of motor and autonomic function in individuals with spinal cord injury. Neuromodulation (2023) 4(23):S1094–7159. doi:10.1016/j.neurom.2023.03.014
234. Wagner, FB, Mignardot, JB, Le Goff-Mignardot, CG, Demesmaeker, R, Komi, S, Capogrosso, M, et al. Targeted neurotechnology restores walking in humans with spinal cord injury. Nature (2018) 563(7729):65–71. doi:10.1038/s41586-018-0649-2
235. Angeli, CA, Boakye, M, Morton, RA, Vogt, J, Benton, K, Chen, Y, et al. Recovery of over-ground walking after chronic motor complete spinal cord injury. N Engl J Med (2018) 379(13):1244–50. doi:10.1056/nejmoa1803588
236. Rowald, A, Komi, S, Demesmaeker, R, Baaklini, E, Hernandez-Charpak, SD, Paoles, E, et al. Activity-dependent spinal cord neuromodulation rapidly restores trunk and leg motor functions after complete paralysis. Nat Med (2022) 28(2):260–71. doi:10.1038/s41591-021-01663-5
237. Lorach, H, Galvez, A, Spagnolo, V, Martel, F, Karakas, S, Intering, N, et al. Walking naturally after spinal cord injury using a brain-spine interface. Nature (2023) 618(7963):126–33. doi:10.1038/s41586-023-06094-5
238. Nam, KY, Kim, HJ, Kwon, BS, Park, JW, Lee, HJ, and Yoo, A. Robot-assisted gait training (Lokomat) improves walking function and activity in people with spinal cord injury: a systematic review. J NeuroEngineering Rehabil (2017) 14(1):24. doi:10.1186/s12984-017-0232-3
239. Yıldırım, MA, Öneş, K, and Gökşenoğlu, G. Early term effects of robotic assisted gait training on ambulation and functional capacity in patients with spinal cord injury. Turk J Med Sci (2019) 49(3):838–43. doi:10.3906/sag-1809-7
240. Liang, Z, Lei, T, Wang, S, Luo, Z, and Hu, X. A simple electrical stimulation cell culture system on the myelination of dorsal root ganglia and Schwann cells. BioTechniques (2019) 67(1):11–5. doi:10.2144/btn-2018-0175
241. James, ND, McMahon, SB, Field-Fote, EC, and Bradbury, EJ. Neuromodulation in the restoration of function after spinal cord injury. Lancet Neurol (2018) 17(10):905–17. doi:10.1016/s1474-4422(18)30287-4
242. Taccola, G, Gad, P, Culaclii, S, Wang, PM, Liu, W, and Edgerton, VR. Acute neuromodulation restores spinally-induced motor responses after severe spinal cord injury. Exp Neurol (2020) 327:113246. doi:10.1016/j.expneurol.2020.113246
243. Houle, JD, Morris, K, Skinner, RD, Garcia-Rill, E, and Peterson, CA. Effects of fetal spinal cord tissue transplants and cycling exercise on the soleus muscle in spinalized rats. Muscle Nerve (1999) 22(7):846–56. doi:10.1002/(sici)1097-4598(199907)22:7<846::aid-mus6>3.0.co;2-i
244. Hutchinson, KJ. Three exercise paradigms differentially improve sensory recovery after spinal cord contusion in rats. Brain (2004) 127(6):1403–14. doi:10.1093/brain/awh160
245. Sandrow-Feinberg, HR, Izzi, J, Shumsky, JS, Zhukareva, V, and Houle, JD. Forced exercise as a rehabilitation strategy after unilateral cervical spinal cord contusion injury. J Neurotrauma (2009) 26(5):721–31. doi:10.1089/neu.2008.0750
246. Vaynman, S, Ying, Z, and Gomez-Pinilla, F. Interplay between brain-derived neurotrophic factor and signal transduction modulators in the regulation of the effects of exercise on synaptic-plasticity. Neuroscience (2003) 122(3):647–57. doi:10.1016/j.neuroscience.2003.08.001
247. Côté, MP, Azzam, GA, Lemay, MA, Zhukareva, V, and Houlé, JD. Activity-dependent increase in neurotrophic factors is associated with an enhanced modulation of spinal reflexes after spinal cord injury. J Neurotrauma (2011) 28(2):299–309. doi:10.1089/neu.2010.1594
248. Ying, Z, Roy, RR, Edgerton, VR, and Gómez-Pinilla, F. Exercise restores levels of neurotrophins and synaptic plasticity following spinal cord injury. Exp Neurol (2005) 193(2):411–9. doi:10.1016/j.expneurol.2005.01.015
249. Gómez-Pinilla, F, Ying, Z, Roy, RR, Molteni, R, and Edgerton, VR. Voluntary exercise induces a BDNF-mediated mechanism that promotes neuroplasticity. J Neurophysiol (2002) 88(5):2187–95. doi:10.1152/jn.00152.2002
250. Marsh, BC, Astill, SL, Utley, A, and Ichiyama, RM. Movement rehabilitation after spinal cord injuries: emerging concepts and future directions. Brain Res Bull (2011) 84(4–5):327–36. doi:10.1016/j.brainresbull.2010.07.011
251. Norrie, BA, Nevett-Duchcherer, JM, and Gorassini, MA. Reduced functional recovery by delaying motor training after spinal cord injury. J Neurophysiol (2005) 94(1):255–64. doi:10.1152/jn.00975.2004
252. Donoghue, JP, Nurmikko, A, Black, M, and Hochberg, LR. Assistive technology and robotic control using motor cortex ensemble-based neural interface systems in humans with tetraplegia. J Physiol (2007) 579(3):603–11. doi:10.1113/jphysiol.2006.127209
253. Wang, W, Collinger, JL, Degenhart, AD, Tyler-Kabara, EC, Schwartz, AB, Moran, DW, et al. An electrocorticographic brain interface in an individual with tetraplegia. PLoS ONE (2013) 8:e55344. doi:10.1371/journal.pone.0055344
254. Nguyen, JS, Su, SW, and Nguyen, HT. Experimental study on a smart wheelchair system using a combination of stereoscopic and spherical vision. In: 2013 35th annual international conference of the IEEE engineering in medicine and biology society (EMBC). Osaka: IEEE (2013). p. 4597–600. Available from: http://ieeexplore.ieee.org/document/6610571/ (Accessed April 12, 2024).
255. Courtine, G, and Bloch, J. Defining ecological strategies in neuroprosthetics. Neuron (2015) 86(1):29–33. doi:10.1016/j.neuron.2015.02.039
256. Van Den Brand, R, Heutschi, J, Barraud, Q, DiGiovanna, J, Bartholdi, K, Huerlimann, M, et al. Restoring voluntary control of locomotion after paralyzing spinal cord injury. Science (2012) 336(6085):1182–5. doi:10.1126/science.1217416
257. Williams, R, and Murray, A. Prevalence of depression after spinal cord injury: a meta-analysis. Arch Phys Med Rehabil (2015) 96(1):133–40. doi:10.1016/j.apmr.2014.08.016
258. Ohry, A. Ethical issues in SCI. Top Spinal Cord Inj Rehabil (2009) 14(3):99–104. doi:10.1310/sci1403-99
259. Samuel, VM, Moses, J, North, N, Smith, H, and Thorne, K. Spinal cord injury rehabilitation: the experience of women. Spinal Cord (2007) 45(12):758–64. doi:10.1038/sj.sc.3102111
260. Ronca, E, Scheel-Sailer, A, Eriks-Hoogland, I, Brach, M, Debecker, I, and Gemperli, A. Factors influencing specialized health care utilization by individuals with spinal cord injury: a cross-sectional survey. Spinal Cord (2021) 59(4):381–8. doi:10.1038/s41393-020-00581-6
261. Fouad, K, Bixby, JL, Callahan, A, Grethe, JS, Jakeman, LB, Lemmon, VP, et al. FAIR SCI ahead: the evolution of the open data commons for pre-clinical spinal cord injury research. J Neurotrauma (2020) 37(6):831–8. doi:10.1089/neu.2019.6674
262. Ross, JS, Lehman, R, and Gross, CP. The importance of clinical trial data sharing: toward more open science. Circ Cardiovasc Qual Outcomes (2012) 5(2):238–40. doi:10.1161/circoutcomes.112.965798
263. Tuszynski, MH, Steeves, JD, Fawcett, JW, Lammertse, D, Kalichman, M, Rask, C, et al. Guidelines for the conduct of clinical trials for spinal cord injury as developed by the ICCP Panel: clinical trial inclusion/exclusion criteria and ethics. Spinal Cord (2007) 45(3):222–31. doi:10.1038/sj.sc.3102009
264. Fawcett, JW, Curt, A, Steeves, JD, Coleman, WP, Tuszynski, MH, Lammertse, D, et al. Guidelines for the conduct of clinical trials for spinal cord injury as developed by the ICCP panel: spontaneous recovery after spinal cord injury and statistical power needed for therapeutic clinical trials. Spinal Cord (2007) 45(3):190–205. doi:10.1038/sj.sc.3102007
265. Lammertse, D, Tuszynski, MH, Steeves, JD, Curt, A, Fawcett, JW, Rask, C, et al. Guidelines for the conduct of clinical trials for spinal cord injury as developed by the ICCP panel: clinical trial design. Spinal Cord (2007) 45(3):232–42. doi:10.1038/sj.sc.3102010
266. Pacheco Barzallo, D, Oña, A, and Gemperli, A. Unmet health care needs and inequality: a cross-country comparison of the situation of people with spinal cord injury. Health Serv Res (2021) 56(S3):1429–40. doi:10.1111/1475-6773.13738
267. Tough, H, Brinkhof, MWG, Siegrist, J, and Fekete, C. Social inequalities in the burden of care: a dyadic analysis in the caregiving partners of persons with a physical disability. Int J Equity Health (2020) 19(1):3. doi:10.1186/s12939-019-1112-1
268. Cardenas, DD, and Jensen, MP. Treatments for chronic pain in persons with spinal cord injury: a survey study. J Spinal Cord Med (2006) 29(2):109–17. doi:10.1080/10790268.2006.11753864
Keywords: spinal cord injury, neuroregeneration, animal models, clinical trials, pathophysiology
Citation: Hassan OI, Takamiya S, Asgarihafshejani A and Fehlings MG (2024) Bridging the gap: a translational perspective in spinal cord injury. Exp. Biol. Med. 249:10266. doi: 10.3389/ebm.2024.10266
Received: 29 May 2024; Accepted: 27 August 2024;
Published: 26 September 2024.
Copyright © 2024 Hassan, Takamiya, Asgarihafshejani and Fehlings. This is an open-access article distributed under the terms of the Creative Commons Attribution License (CC BY). The use, distribution or reproduction in other forums is permitted, provided the original author(s) and the copyright owner(s) are credited and that the original publication in this journal is cited, in accordance with accepted academic practice. No use, distribution or reproduction is permitted which does not comply with these terms.
*Correspondence: Michael G. Fehlings, bWljaGFlbC5mZWhsaW5nc0B1aG4uY2E=